the Creative Commons Attribution 4.0 License.
the Creative Commons Attribution 4.0 License.
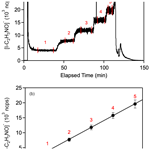
Calibration of hydroxyacetonitrile (HOCH2CN) and methyl isocyanate (CH3NCO) isomers using I− chemical ionization mass spectrometry (CIMS)
Zachary Finewax
Aparajeo Chattopadhyay
J. Andrew Neuman
James M. Roberts
James B. Burkholder
The toxic reduced nitrogen compound methyl isocyanate (CH3NCO, MIC) has been reported as present in wildfire and biomass burning emissions, agricultural fumigation plumes, and indoor air. Its isomer, hydroxyacetonitrile (HOCH2CN, glycolonitrile, or HAN) has not been observed in the Earth's atmosphere to date. In this study, absolute sensitivity calibrations for these isomers using two I− chemical ionization mass spectrometry (I-CIMS) instruments, the time-of-flight (ToF) and quadrupole (Quad) instruments, commonly used in laboratory and field measurements, were performed, for the first time, over a range of ion-molecule reactor temperatures (10–40 °C) and I(H2O)− I− ratio (0.01–1). This study demonstrates that I-CIMS, under typical operating conditions, is not sensitive to MIC with limits of detection (LOD) of > 860 and > 570 ppb for the ToF and Quad I-CIMS instruments, respectively. Both I-CIMS instruments are, however, highly sensitive to the HAN isomer with 0.3 and 3 ppt LODs for the ToF-CIMS and Quad-CIMS instruments, respectively. The present results show that several recent field studies using I-CIMS instrument detection have misattributed the C2H3NO signal to MIC. This study proposes that HAN rather than MIC was most likely the C2H3NO isomer observed in those field studies, although the source chemistry for HAN remains uncharacterized. This study demonstrates the importance of applying absolute calibration standards in the identification and quantification of isomeric compounds.
- Article
(502 KB) - Full-text XML
- BibTeX
- EndNote
The identification and quantitative measurement of atmospheric trace species are critical elements in the development of air quality models and the establishment of environmental policies. Methyl isocyanate (CH3NCO, MIC) and hydroxyacetonitrile (HOCH2CN, glycolonitrile, or HAN) (Wolfsie, 1960) are toxic reduced nitrogen isomers present in an extreme range of environments. MIC has been reported in wildfire and biomass burning, e.g., Koss et al. (2018); building fire emissions (Blomqvist et al., 2003); agricultural fumigation plumes (Woodrow et al., 2014); and indoor air (Bekki et al., 2018), particularly where there is cigarette smoke (Moldoveanu, 2010), cooking emissions, and chlorine-disinfectant use. HAN has been observed spectroscopically in interstellar space (see Zhao et al., 2021, and references within), while measurements in the Earth's atmosphere have not been reported to date. The atmospheric degradation of MIC leads to the formation of isocyanic acid (HNCO) (Papanastasiou et al., 2020), another toxic reduced nitrogen compound. The atmospheric chemistry of the HAN isomer is not presently characterized.
The implementation of I− chemical ionization mass spectrometry (I-CIMS) instruments, in particular high-resolution time-of-flight mass spectrometer instruments (I-CIMS ToF-MS), has enabled high-sensitivity laboratory and ground-based field measurements, as well as high spatial and temporal resolution in airborne field studies. However, a general limitation of CIMS measurements is the inability to distinguish isomers, e.g., MIC and HAN C2H3NO isomers, without using pre-separation techniques such as gas chromatography. Field measurements of MIC have been reported in outdoor (Priestley et al., 2018) and indoor (Mattila et al., 2020a, b; Wang et al., 2022) environments using (I-CIMS) instruments, with sensitivity calibration estimated using voltage-scan techniques (Mattila et al., 2020b; Wang et al., 2022) and analogy to the sensitivity of other volatile organic compound (VOC) species (Priestley et al., 2018).
The primary objective of this work was to establish sample handling and I-CIMS sensitivity calibration protocols for the C2H3NO MIC and HAN isomers. Absolute pressure and FTIR measurements were used to establish MIC standards, while diffusion and infusion methods coupled with total reactive nitrogen (Nr) methods were used for the quantification of HAN standards. Calibration measurements are presented for I-CIMS time-of-flight (ToF-CIMS) and quadrupole CIMS (Quad-CIMS) mass spectrometry instruments over a range of I(H2O)− I− ratios and ion-molecule reactor (IMR) temperatures. The results from this work can be applied to similar instruments used in laboratory and field studies, although instrument sensitivities may vary somewhat depending on instrument configuration and operating conditions. This work demonstrates that the recent field measurements reported by Priestley et al. (2018), Mattila et al. (2020a), and Wang et al. (2022) have misattributed their measured I-CIMS signal, which was assigned to MIC when it was most likely due to HAN.
A primary goal of this work was the development of measurement protocols for hydroxyacetontrile (HOCH2CN, HAN) and methyl isocyanate (CH3NCO, MIC) using iodide chemical ionization mass spectrometry (I-CIMS) with time-of-flight mass spectrometer (ToF-CIMS) (Lee et al., 2014) and quadrupole mass spectrometer (Quad-CIMS) (Neuman et al., 2000) instruments. We have focused on calibration measurements for ToF-CIMS and Quad-CIMS instruments for which calibrations for MIC and HAN have not been performed previously. An emphasis in this work was placed on MIC and HAN sample handling and quantification, i.e., knowing what the sample concentration is at the instrument inlet.
MIC and HAN samples for calibration measurements were prepared using dilute gas mixtures prepared using absolute pressure measurements, diffusion sources, and infusion sources that are described in detail below. Absolute concentrations were determined using Fourier transform infrared spectroscopy (FTIR) spectroscopy (MIC) and a total reactive nitrogen (Nr) instrument (HAN). Calibration measurements were performed with ToF- and Quad-CIMS instruments to evaluate differences in sensitivity for instruments with different configurations, e.g., ion-molecule reactor (IMR) geometries, ionization sources, and ion focusing optics. In addition, sensitivities were determined for a range of IMR temperatures (10–40 °C) and I(H2O)− I− ratios commonly used in laboratory and field studies, which are known to affect I-CIMS sensitivity (see Robinson et al., 2022). The MIC and HAN calibration methods, Nr instrument and methods, and ToF- and Quad-CIMS instruments are described below.
2.1 Methyl isocyanate source and calibration
Methyl isocyanate (MIC) is a stable volatile liquid at room temperature, with a vapor pressure of ∼ 467 hPa (1 hPa = 0.75 Torr) at 25 °C. For the present study, an MIC sample was obtained commercially in pure form. Dilute gas-phase methyl isocyanate (MIC) samples were prepared manometrically at a total pressure of 1066 hPa in a 12 L Pyrex bulb with He bath gas. The MIC mixing ratio was ∼ 3.5 %. The MIC mixing ratio was also determined by Fourier transform infrared spectroscopy using the absorption cross section data measured in Papanastasiou et al. (2020) from our laboratory. Dilute high-pressure gas standards were prepared by diluting glass bulb standards into aluminum cylinders pressurized with zero air. Methyl isocyanate bulb and aluminum cylinder standards were quantitatively added to the zero-air flow sampled by the I-CIMS instruments.
2.2 Hydroxyacetonitrile (HAN) source and calibration methods
HAN is a stable semi-volatile compound, with a vapor pressure of 1.33 hPa at 336 K (NIOSH) that is available commercially as a 70 wt % mixture with H2O. Over the course of this study, liquid-to-gas-phase diffusion and infusion methods were applied for the delivery of gas-phase HAN to the Nr calibration and I-CIMS instruments.
The diffusion source is described in detail elsewhere (Roberts et al., 2010; Williams et al., 2000). Basically, a capillary diffusion cell regulated vapor from the liquid sample into the gas stream feeding into the Nr and I-CIMS instruments. The concentration of the source compound is regulated by varying the total gas flow, i.e., dilution factor. This source method requires an independent determination of the compound concentration in the gas stream. The infusion method has been used previously in our laboratory and is described in detail elsewhere (Bernard et al., 2017, 2018). Basically, a syringe pump is used to deliver a constant liquid flow (0.01–0.3 µL min−1 in our experiments) of a HAN sample into the gas stream feeding into the Nr and I-CIMS instruments. The region of the liquid–gas interface was heated (40 to 60 °C) to ensure uniform volatilization and minimize potential sample condensation. Measurements were performed using the commercially available stock HAN solution and a HAN sample diluted in acetone, prepared offline. The mixing ratio of HAN in the gas flow can in principle be calculated using the calibrated gas and liquid flow rates, the density of the compound, and its liquid mixing ratio, although in this work, the HAN infusion source was calibrated using a total reactive nitrogen, Nr, measurement.
2.3 Total reactive nitrogen, Nr, measurement
The infusion source gas-phase concentration of HAN was determined by a total reactive nitrogen, Nr, measurement. Total reactive nitrogen is defined as all reduced and oxidized nitrogen-containing compounds with the exception of N2 and N2O. The Nr instrument has been demonstrated for both gas-phase and particle-phase Nr and is described in detail elsewhere (Stockwell et al., 2018). In this study, the nitrogen-containing compounds in a gas-phase sample are first catalytically converted on a 750 °C Pt catalyst to NO and NO2. The NO2 is subsequently converted to NO on a molybdenum oxide catalyst at 350 °C. The NO then reacts with an excess of O3 to form NO2, which is detected by chemiluminescence (Williams et al., 1998). The Nr instrument was calibrated using commercial dilute mixtures of NO (5.18 ppm) and HCN (9.5 ppm) in an N2 bath gas. HCN and NO calibrations performed over the course of the study agreed to within 3 %. The total flow through the Nr instrument was set to 1.048 SLPM (standard liter per minute) and the total zero-air flow from the infusion source was 2.148 SLPM. The excess flow passed through an exhaust line. Considering uncertainties in the standards and flow rates, the 2σ uncertainty in the Nr calibration of the HAN source was estimated to be 15 %. The HAN infusion source was calibrated by measuring the Nr concentration as a function of the liquid injection flow rate. Over the course of the study, multiple calibration experiments were performed using independently prepared HAN samples.
2.4 Iodide chemical ionization mass spectrometry (I-CIMS)
I-CIMS has the ability to measure sub-part per trillion (ppt) gas-phase concentrations of organic acids, halogens, oxidized organic compounds, and N2O5 at up to a 10 Hz resolution (Huey, 2007; Neuman et al., 2000; Veres et al., 2020). This relatively soft ionization technique usually yields an I− cluster ion with the intact analyte molecule. I-CIMS is highly selective, with the sensitivity to an analyte dependent on the binding enthalpy of the compound with I−. Time-of-flight mass spectrometers typically contain ion focusing quadrupoles that can also impart changes to the sensitivity of analytes due to mass-dependent ion transmission and collision-induced dissociation (Robinson et al., 2022). The I-CIMS instruments used in the present study have been described in detail previously, and only pertinent details are described below.
2.4.1 Time-of-flight chemical ionization mass spectrometer (ToF-CIMS)
The ToF-CIMS is set up with a pressure-controlled inlet, ion molecule reactor (IMR), small-segmented quadrupole (SSQ), big-segmented quadrupole (BSQ), and time-of-flight (ToF) mass analyzer (Veres et al., 2020). A Kr lamp provided vacuum ultraviolet radiation at 124 and 117 nm that photoionized CH3I to produce I− in the IMR (Breitenlechner et al., 2022; Ji et al., 2020). Analytes (A) react with I− or I(H2O)− to form adducts:
with I-C2H3NO− detected at = 183.9265. Key instrument conditions that impact the sensitivity for an analyte are the IMR temperature and I(H2O)− I− ratio (Lee et al., 2014; Robinson et al., 2022; Veres et al., 2020). In this study, the IMR was temperature controlled between 20 and 40 °C, and the I(H2O)− I− ratio was dynamically controlled at 0.01, i.e., dry and over the range 0.4 to 0.6, which represents a typical range of operating conditions for laboratory and field instruments. The ToF-CIMS inlet was pressure controlled at 130 hPa, and the flow into the inlet was controlled to 6 SLPM. The IMR was pressure controlled to 44 hPa (Zhang and Zhang, 2021). The pressure in the SSQ and BSQ were 1.7 and 0.013 hPa, respectively. The sum of I I(H2O)− was typically 5 MHz in these experiments.
2.4.2 Quadrupole chemical ionization mass spectrometer (Quad-CIMS)
The Quad-CIMS instrument setup consisted of a critical orifice inlet (600 µm diameter) combined with an IMR, ion focusing lenses, and a quadrupole mass analyzer (Neuman et al., 2000). I− ions were generated by passing a 0.1 % CH3I in N2 mixture through a 210Po radioactive source. The IMR was temperature controlled between 10 and 30 °C. The I(H2O)− I− ratio was adjusted between 0.01 and 1.0 by controlling the humidity of the gas flow into the CIMS, which ranged from 4.2 to 4.5 SLPM. This system did not have an intermediate-pressure zone between the IMR and mass spectrometer chamber, common to many I-CIMS instruments, that would serve as a collisional-dissociation chamber. The pressure in the IMR was maintained at 37 hPa by varying the pumping speed. The mass resolution of the Quad-CIMS instrument is ∼ 200 and that of the ToF-CIMS is ∼ 5000. The sum of I I(H2O)− was typically 500 kHz in these experiments.
2.5 Materials
Synthetic air (zero grade), N2 (UHP, 99.999 %), He (UHP, 99.999 %), and CH3I (99 %) and acetone (99 %) were used as provided. Standard dilute mixtures of NO (5.18 ppm in N2) and HCN (9.5 ppm in N2) were obtained commercially. Hydroxyacetonitrile (HAN, ∼ 70 % in H2O; CAS RN – 107-16-4) and methyl isocyanate (MIC, 97+ %; CAS RN – 624-83-9) samples were obtained commercially. The MIC sample contained a < 3 % trimethylchlorosilane (CAS RN – 75-77-4) inhibitor. The HAN and MIC samples were degassed in freeze–pump–thaw cycles. Samples were stored in a chemical refrigerator in vacuum-sealed Pyrex reservoirs prior to use.
A dilute (3.48 %) gas mixture of MIC in He was prepared manometrically in a 12 L Pyrex bulb. Fourier transform infrared spectroscopy (FTIR) measurements (1 cm−1 resolution, 425 cm pathlength) using a previously determined absorption spectrum from our laboratory (Papanastasiou et al., 2020) confirmed the mixing ratio to within 10 % of the manometric preparation. Standard dilute solutions of HAN in acetone were prepared volumetrically using 1.0–2.0 µL of the commercial HAN solution and 5.0 or 10.0 mL of acetone. The standard solutions were stored in a chemical refrigerator in vacuum-sealed Pyrex reservoirs. Samples used in the calibration infusion experiments were extracted from the standard solution with a gas-tight 10 or 100 µL syringe.
3.1 Methyl isocyanate (CH3NCO, MIC)
ToF-CIMS and Quad-CIMS measurements with MIC mixing ratios of up to 860 ppb yielded no measurable signal for the I-MIC− or I(H2O)-MIC− adducts above the background (1σ background noise level of 3 ncps). Measurements were performed over the range of CIMS conditions described in the experimental section. The sensitivity upper limits obtained are given in Table 1 and represent the signal increase (normalized to 1 million counts per second (cps) of the sum of I I(H2O)− per mixing ratio unit increase of the compound). Our measurements indicate that MIC mixing ratios of greater than 1 ppm may be required to generate a detectable I-CIMS signal if MIC can be detected by I-CIMS at all.
Table 1ToF-CIMS and Quad-CIMS instrument sensitivity (S) and limits of detection (LOD) measured in this work for hydroxyacetonitrile (HOCH2CN, HAN) and methyl isocyanate (CH3NCO, MIC) for typical field operating conditions with an IMR temperature of 30 °C, I(H2O)− I− ratio of 0.55, and 1 s integration. See Fig. 3 for sensitivity dependence on operating conditions.
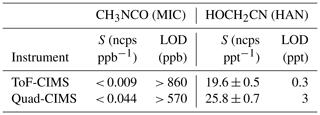
The ToF- and Quad-CIMS instruments used in the present study were found to be insensitive to the detection of CH3NCO (MIC). The lack of MIC sensitivity for both instruments suggests that the I− cluster with MIC is not thermodynamically stable in the IMR. The ion focusing or ion optics in the ToF-CIMS and the Quad-CIMS are, however, quite different. The ToF-CIMS SSQ was set to 1.7 hPa, so collisions occur in this region, resulting in some collisional dissociation. The BSQ focuses ions and can also result in fragmentation of I− clusters. The ion optics of the Quad-CIMS use only static electric fields in a low-pressure region (< hPa) and do not have sufficient frequency to dissociate I− clusters. Given the lack of sensitivity of both ToF- and Quad-CIMS instruments to MIC, it is unlikely that other common I-CIMS instrument configurations have the sensitivity to detect MIC at atmospherically relevant mixing ratios.
3.2 Hydroxyacetonitrile (HOCH2CN, HAN)
The diffusion source, with the commercial 70 % HAN/H2O solution, proved to be an unsuccessful HAN delivery method. This method produced high gas-phase concentrations of an HCN impurity as identified by I-CIMS. Although HCN was a minor sample impurity, < 1 %, its high vapor pressure (862 hPa at 295 K; Perry and Porter, 1926) and low Henry's law coefficient (∼ 9 M Atm−1; Burkholder et al., 2019), resulted in a [HCN]/[HAN] gas-phase mixing ratio of greater than 1000; i.e., HAN was not detectable by I-CIMS using this source. The high gas-phase HCN concentration using the diffusion method precluded quantitative calibration of HAN concentration using the total reactive nitrogen calibration method.
The infusion source did not produce detectable gas-phase HCN above the detection limit of the mass spectrometers; i.e., the HCN impurity level was much less than 1 %. Therefore, HCN did not influence the absolute HAN calibration determination using the Nr instrument. The region around the infusion source was heated to 50 °C when using the commercially available HAN stock solution. Although this source worked, it did not provide a stable HAN signal to within 10 %. This source, using a dilute HAN/acetone mixture with the region of the infusion source heated to 45 °C, i.e., slightly below the boiling point of acetone, yielded more stable HAN signals with variations of a few percent. Measurements performed at temperatures greater than 45 °C yielded reasonable results, but the signal was less stable, most likely due to boiling of the acetone solvent.
Calibration of hydroxyacetonitrile (HAN) solutions by total reactive nitrogen (Nr) is shown in Fig. 1. The infusion method produces a stable source of HAN with a high signal-to-noise ratio for a typical calibration experiment. The total HAN concentration was set by adjusting the injection flow rate. Individual solutions were calibrated multiple times, as shown in Fig. 1. The 2σ uncertainty in the fit precision of [HAN] vs. the infusion flow rate was determined to be 4 %. The small positive intercept may be due to minor unidentified Nr impurities.
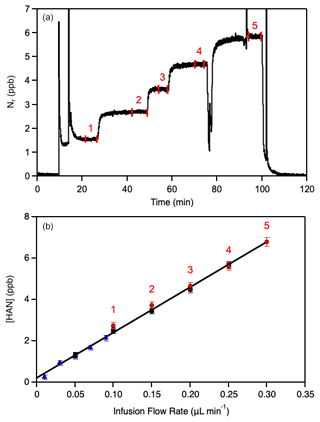
Figure 1Calibration of the hydroxyacetonitrile (HOCH2CN, HAN) infusion source at 23 °C using the total nitrogen, Nr, instrument (see text for details of the Nr instrument). (a) Background-corrected time series of a representative calibration experiment. The data within the numbered vertical lines were averaged and correspond to the numbered points in (b). (b) Calibration of the HAN mixing ratio taking the Nr-measured mixing ratio, example in (a), to be equal to the HAN mixing ratio as a function of the infusion source flow rate. Different symbols represent independent calibration experiments. The line is an unweighted linear least-squares fit of all the data. Error bars represent 2σ measurement precision.
Calibrations of the HAN signal on the ToF-CIMS and Quad-CIMS instruments were made by varying the infusion flow rate; see representative data for the Quad-CIMS in Fig. 2. The obtained ToF- and Quad-CIMS sensitivity for HAN given in Table 1 was obtained using the infusion method with the dilute HAN/acetone samples. For typical field operating conditions with an IMR temperature of 30 °C, I(H2O)− I− ratio of 0.55, and 1 s integration, the HAN sensitivity of the ToF I-CIMS was determined to be ∼ 20 ncps ppt−1.
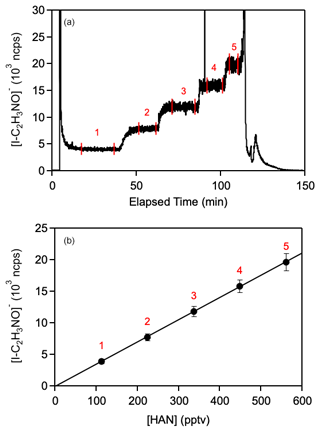
Figure 2Calibration of the Quad-CIMS instrument using the hydroxyacetonitrile (HOCH2CN, HAN) infusion source at 23 °C (see text for details of the Quad-CIMS instrument). (a) Background-corrected time series of a representative Quad-CIMS calibration experiment (IMR temperature = 20 °C; I(H2O)− I). The data within the numbered vertical lines were averaged and correspond to the numbered points in (b). (b) Quad-CIMS HAN calibration curve where the HAN concentration was determined from the Nr calibration of the infusion source, e.g., see Fig. 1. The line is an unweighted linear least-squares fit of the data. Error bars represent 2σ measurement precision.
The HAN concentration from the infusion source at the instrument inlet was varied for each IMR temperature and I(H2O)− I− ratio to determine the HAN sensitivity temperature and I(H2O)− I− dependence shown in Fig. 3. The ToF-CIMS and Quad-CIMS instruments are both highly sensitive to HAN but displayed slightly different temperature and I(H2O)− I− dependencies. As the temperature increased, the HAN sensitivity decreased, consistent with the [I-HAN]− adduct being less stable at higher temperatures. For the ToF-CIMS instrument, the HAN sensitivity decreased by a factor of ∼ 1.5–2 between 20 and 40 °C at the highest I(H2O)− I− ratio included in this study. For the Quad-CIMS instrument, a ∼ 25 % decrease in HAN sensitivity was observed at an I(H2O)− I− ratio of 1 when increasing the IMR temperature from 10 to 30 °C. The I(H2O)− I− dependency for HAN sensitivity was fit reasonably well with a quadratic dependence on I(H2O)− I−, A + B(I(H2O)− I−) – C(I(H2O)− I−)2, where the constant term represents HAN clustering with I−, Reaction (1); the linear term represents HAN undergoing a ligand switching reaction with I(H2O)−, Reaction (2); and the quadratic term may represent a lack of reactivity of higher-order H2O clusters, I(H2O), where n>1 or a shift in the
equilibrium.
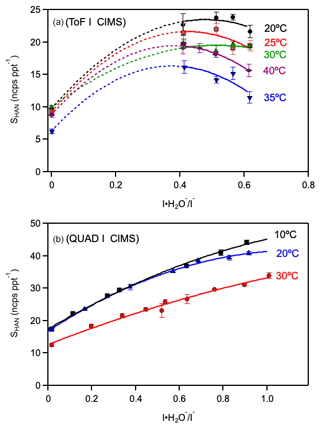
Figure 3Hydroxyacetonitrile (HOCH2CN, HAN) calibration factors for the ToF and Quad mass spectrometers used in this work as a function of the I(H2O)− I− ratio and ion-molecule reactor (IMR) temperature. Error bars represent 2σ precision of the linear calibration fits. Lines are empirical polynomial fits to guide the eye. (a) HAN calibration factors measured for the ToF-CIMS. Symbols represent IMR temperatures of 20 °C (black circles), 25 °C (red squares), 30 °C (green triangles), 35 °C (blue upside-down triangles), and 40 °C (purple diamonds). (b) Quad-CIMS HAN calibration data. Symbols represent IMR temperatures of 10 °C (black squares), 20 °C (blue triangles), and 30 °C (red circles).
There is a plausible explanation for the differences in the Quad-CIMS and ToF-CIMS instrument I(H2O)− I− sensitivity dependence. First, there are differences in these instruments in the ion focusing downstream of the IMR. The ToF-CIMS contains an SSQ at 1.73 hPa that imparts an electric field and acts as a collisional dissociation chamber. The subsequent BSQ also imparts an electric field that can dissociate weakly bound I− clusters. The I(H2O)− I− is dynamically controlled in the ToF-CIMS by what is observed at the detector. Therefore, the I(H2O)− (and I(H2O) ion counts are not representative of the I(H2O)− I− ratio in the IMR; i.e., the I(H2O)− I− ratio in the IMR is being underestimated, although we were unable to quantify the dependence. The Quad-CIMS, which has ion lenses at low pressure downstream of the IMR, 1.3–0.13 × 10−3 hPa, should have only minor collisional dissociation, if any. Therefore, the I(H2O)− I− ratio measured by the Quad-CIMS is, most likely, close to the actual I(H2O)− I− in the IMR. In conclusion, it is recommended that instruments used to quantify HAN be calibrated under actual operating conditions. The protocols presented in this work can be used for the calibration of HAN.
In this study, protocols for determining I-CIMS instrument sensitivity for CH3NCO (MIC) and HOCH2CN (HAN), two stable toxic C2H3NO isomers (Panda et al., 2023), were developed. Calibration of our ToF-CIMS and Quad-CIMS instruments for HAN were performed over a range of instrument conditions commonly used in laboratory and field experiments, including ion molecule reactor (IMR) temperature (10–40 °C) and I(H2O)− I− ratios between 0.1 and 1. Both I-CIMS instruments were found to be highly sensitive to HAN, with 0.3 and 3 ppt limits of detection (LOD) for the ToF-CIMS and Quad-CIMS instruments, respectively, for measurements with the IMR at 30 °C, I(H2O)− I− ratio of 0.55, and 1 s integration (see Table 1). The instruments were insensitive to MIC, with LODs of > 860 and > 570 ppb for the ToF and Quad I-CIMS instruments, respectively. A weak negative temperature dependence and systematic positive I(H2O)− I− ratio dependence was observed for HAN. The ToF and Quad I-CIMS instruments have similar normalized sensitivities, reflecting the similar chemistry in the ion molecule reaction regions. The ToF instrument has much lower LOD because the reagent ion concentration was approximately 10 times greater than that in the Quad instrument, and the ToF has far better mass resolution. The results from this study should, to a first approximation, translate to other laboratory and field I-CIMS instruments.
Our work demonstrates that the previous field studies of Priestley et al. (2018), Mattila et al. (2020a, b), and Wang et al. (2022), which used I-CIMS detection methods, misattributed the C2H3NO mass signal as methyl isocyanate (MIC) and also provides evidence for the observation of HAN, a previously unrecognized species in these environments. Our results suggest that HAN was observed but do not imply that MIC was not present in the environments studied by Priestley et al. (2018), Mattila et al. (2020a, b), and Wang et al. (2022). Since I-CIMS is not sensitive to MIC, alternative measurement methods, such as proton transfer CIMS, would be required to identify the presence of MIC. Our work indicates that HAN is likely to be present in the troposphere. Iyer et al. (2016) and Hyttinen et al. (2018) provide an explanation for the significant difference in the I-CIMS sensitivity for MIC (CH3NCO) and HAN (HOCH2CN) due to the I-cluster binding energies. That is, the H-bonding with the HO group in HAN leads to a stable I-cluster, while MIC would not form a stable I-cluster.
The heterogeneous and gas-phase atmospheric chemistry of HOCH2CN (HAN) are, however, not presently characterized. Here, we postulate that in addition to primary HAN emissions, e.g., from wildfires, HAN would be formed heterogeneously in clouds or on hydrated aerosol via the liquid-phase reaction:
The partitioning of HAN between the liquid and gas phases will depend on its Henry's law coefficient, which has not been measured to date. Sander (2023) reports an estimated Henry's law coefficient value of ∼ 130 M atm−1, using a quantitative structure–property relationship, which implies the partitioning of HAN into the gas phase. HCN and H2CO are ubiquitous in the atmosphere, with elevated concentrations in wildfire plumes, which may lead to significant enhancement in the HAN concentration following wildfire plume exposure to clouds. Our work will aid future laboratory and field studies to identify the atmospheric source chemistry of HAN as well as its atmospheric loss processes and degradation products.
No data sets were used in this article.
ZF undertook the experimental measurements and contributed to the first draft and writing of the paper. AC performed the MIC infrared measurements and initial ToF MIC measurements. JAN performed the initial ToF MIC measurements. JMR performed the initial Nr measurements and contributed to the writing of the paper. JBB supervised the project and completed the writing of the paper.
The contact author has declared that none of the authors has any competing interests.
Publisher’s note: Copernicus Publications remains neutral with regard to jurisdictional claims made in the text, published maps, institutional affiliations, or any other geographical representation in this paper. While Copernicus Publications makes every effort to include appropriate place names, the final responsibility lies with the authors.
This research was supported in part by the NOAA Climate Goal and NASA Atmospheric Composition Programs.
This paper was edited by Fred Stroh and reviewed by Delphine Farmer and John Crounse.
Bekki, K., Uchiyama, S., and Kunugita, N.: Analysis of isocyanates in indoor dust, Anal. Bioanal. Chem., 410, 4247–4251, https://doi.org/10.1007/s00216-018-1110-y, 2018.
Bernard, F., Papanastasiou, D. K., Papadimitriou, V. C., and Burkholder, J. B.: Infrared absorption spectra of linear (L2-L5) and cyclic (D3-D6) permethylsiloxanes, J. Quant. Spectros. Rad. Trans., 202, 247–254, https://doi.org/10.1016/j.jqsrt.2017.08.006, 2017.
Bernard, F., Papanastasiou, D. K., Papadimitriou, V. C., and Burkholder, J. B.: Infrared absorption spectra of N(CxF2x+1)3, x = 2–5 perfluoroamines, J. Quant. Spectrosc. Rad. Trans., 211, 166-171, https://doi.org/10.1016/j.jqsrt.2018.02.039, 2018.
Blomqvist, P., Hertzberg, T., Dalene, M., and Skarping, G.: Isocyanates, aminoisocyanates and amines from fires – a screening of common materials found in buildings, Fire Mater., 27, 275–294, https://doi.org/10.1002/fam.836, 2003.
Breitenlechner, M., Novak, G. A., Neuman, J. A., Rollins, A. W., and Veres, P. R.: A versatile vacuum ultraviolet ion source for reduced pressure bipolar chemical ionization mass spectrometry, Atmos. Meas. Tech., 15, 1159–1169, https://doi.org/10.5194/amt-15-1159-2022, 2022.
Burkholder, J. B., Sander, S. P., Abbatt, J., Barker, J. R., Cappa, C., Crounse, J. D., Dibble, T. S., Huie, R. E., Kolb, C. E., Kurylo, M. J., Orkin, V. L., Percival, C. J., Wilmouth, D. M., and Wine, P. H.: “Chemical Kinetics and Photochemical Data for Use in Atmospheric Studies, Evaluation No. 19,” JPL Publication 19-5, Jet Propulsion Laboratory, Pasadena, 2020 http://jpldataeval.jpl.nasa.gov (last access: May 2024), 2019.
Huey, L. G.: Measurement of trace atmospheric species by chemical ionization mass spectrometry: speciation of reactive nitrogen and future directions, Mass Spectrom. Rev., 26, 166–184, https://doi.org/10.1002/mas.20118, 2007.
Hyttinen, N., Otkjaer, R. V., Iyer, S., Kjaergaard, H. G., Rissanen, M. P., Wennberg, P. O., and Kurten, T.: Computational comparison of different reagent ions in the chemical ionization of oxidized multifunctional compounds, J. Phys. Chem. A., 122, 269–279, https://doi.org/10.1021/acs.jpca.7b10015, 2018.
Iyer, S., Lopez-Hilfiker, F., Lee, B. H., Thornton, J. A., and Kurten, T.: Modeling the detection of organic and inorganic compounds using iodide-based chemical ionization, J. Phys. Chem. A, 120, 576–587, https://doi.org/10.1021/acs.jpca.5b09837, 2016.
Ji, Y., Huey, L. G., Tanner, D. J., Lee, Y. R., Veres, P. R., Neuman, J. A., Wang, Y., and Wang, X.: A vacuum ultraviolet ion source (VUV-IS) for iodide–chemical ionization mass spectrometry: a substitute for radioactive ion sources, Atmos. Meas. Tech., 13, 3683–3696, https://doi.org/10.5194/amt-13-3683-2020, 2020.
Koss, A. R., Sekimoto, K., Gilman, J. B., Selimovic, V., Coggon, M. M., Zarzana, K. J., Yuan, B., Lerner, B. M., Brown, S. S., Jimenez, J. L., Krechmer, J., Roberts, J. M., Warneke, C., Yokelson, R. J., and de Gouw, J.: Non-methane organic gas emissions from biomass burning: identification, quantification, and emission factors from PTR-ToF during the FIREX 2016 laboratory experiment, Atmos. Chem. Phys., 18, 3299–3319, https://doi.org/10.5194/acp-18-3299-2018, 2018.
Lee, B. H., Lopez-Hilfiker, F. D., Mohr, C., Kurten, T., Worsnop, D. R., and Thornton, J. A.: An iodide-adduct high-resolution time-of-flight chemical-ionization mass spectrometer: Application to atmospheric inorganic and organic compounds, Environ. Sci. Technol., 48, 6309–6317, https://doi.org/10.1021/es500362a, 2014.
Mattila, J. M., Arata, C., Wang, C., Katz, E. F., Abeleira, A., Zhou, Y., Zhou, S., Goldstein, A. H., Abbatt, J. P. D., DeCarlo, P. F., and Farmer, D. K.: Dark chemistry during bleach cleaning enhances oxidation of organics and secondary organic aerosol production indoors, Environ. Sci. Tech. Lett., 7, 795–801, https://doi.org/10.1021/acs.estlett.0c00573, 2020a.
Mattila, J. M., Lakey, P. S. J., Shiraiwa, M., Wang, C., Abbatt, J. P. D., Arata, C., Goldstein, A. H., Ampollini, L., Katz, E. F., DeCarlo, P. F., Zhou, S., Kahan, T. F., Cardoso-Saldaña, F. J., Ruiz, L. H., Abeleira, A., Boedicker, E. K., Vance, M. E., and Farmer, D. K.: Multiphase chemistry controls inorganic chlorinated and nitrogenated compounds in indoor air during bleach cleaning, Environ. Sci. Tech., 54, 1730–1739, https://doi.org/10.1021/acs.est.9b05767, 2020b.
Moldoveanu, S. C.: Analysis of acrylonitrile and α-methacrylonitrile in vapor phase of mainstream cigarette smoke using a charcoal trap for collection, Contrib. Tobac. Res., 24, 145–156, https://doi.org/10.2478/cttr-2013-0892, 2010.
Neuman, J. A., Gao, R. S., Schein, M. E., Ciciora, S. J., Holecek, J. C., Thompson, T. L., Winkler, R. H., McLaughlin, R. J., Northway, M. J., Richard, E. C., and Fahey, D. W.: A fast-response chemical ionization mass spectrometer for in situ measurements of HNO3 in the upper troposphere and lower stratosphere, Rev. Sci. Instrum., 71, 3886–3894, https://doi.org/10.1063/1.1289679, 2000.
NIOSH (National Institute for Occupational Safety and Health): Glycolonitrile National Institute Occupational Safety Health, https://www.cdc.gov/niosh/npg/npgd0304.html (last access: May 2024), 2024.
Panda, S., Chiranjibi, A., Awasthi, D., Ghoshal, S., and Anoop, A.: Exploring the chemical space of C2H3NO isomers and bimolecular reactions with hydrogen cyanide and formaldehyde: Insights into the emergence of life, Earth Space Chem., 7, 1739–1752, https://doi.org/10.1021/acsearthspacechem.3c00113, 2023.
Papanastasiou, D. K., Bernard, F., and Burkholder, J. B.: Atmospheric fate of methyl isocyanate, CH3NCO: OH and Cl reaction kinetics and identification of formyl isocyanate, HC(O)NCO, Earth Space Chem., 4, 1626–1637, https://doi.org/10.1021/acsearthspacechem.0c00157, 2020.
Perry, J. H. and Porter, F.: The vapor pressures of solid and liquid hydrogen cyanide, J. Amer. Chem. Soc., 48, 297–300, 1926.
Priestley, M., Le Breton, M., Bannan, T. J., Leather, K. E., Bacak, A., Reyes-Villegas, E., De Vocht, F., Shallcross, B. M. A., Brazier, T., Khan, M. A., Allan, J., Shallcross, D. E., Coe, H., and Percival, C. J.: Observations of isocyanate, amide, nitrate, and nitro compounds from an anthropogenic biomass burning using a Tof-CIMS, J. Geophys. Res., 123, 7687–7704, https://doi.org/10.1002/2017jd027316, 2018.
Roberts, J. M., Veres, P., Warneke, C., Neuman, J. A., Washenfelder, R. A., Brown, S. S., Baasandorj, M., Burkholder, J. B., Burling, I. R., Johnson, T. J., Yokelson, R. J., and de Gouw, J.: Measurement of HONO, HNCO, and other inorganic acids by negative-ion proton-transfer chemical-ionization mass spectrometry (NI-PT-CIMS): application to biomass burning emissions, Atmos. Meas. Tech., 3, 981–990, https://doi.org/10.5194/amt-3-981-2010, 2010.
Robinson, M. A., Neuman, J. A., Huey, L. G., Roberts, J. M., Brown, S. S., and Veres, P. R.: Temperature-dependent sensitivity of iodide chemical ionization mass spectrometers, Atmos. Meas. Tech., 15, 4295–4305, https://doi.org/10.5194/amt-15-4295-2022, 2022.
Sander, R.: Compilation of Henry's law constants (version 5.0.0) for water as solvent, Atmos. Chem. Phys., 23, 10901–12440, https://doi.org/10.5194/acp-23-10901-2023, 2023.
Stockwell, C. E., Kupc, A., Witkowski, B., Talukdar, R. K., Liu, Y., Selimovic, V., Zarzana, K. J., Sekimoto, K., Warneke, C., Washenfelder, R. A., Yokelson, R. J., Middlebrook, A. M., and Roberts, J. M.: Characterization of a catalyst-based conversion technique to measure total particulate nitrogen and organic carbon and comparison to a particle mass measurement instrument, Atmos. Meas. Tech., 11, 2749–2768, https://doi.org/10.5194/amt-11-2749-2018, 2018.
Veres, P. R., Neuman, J. A., Bertram, T. H., Assaf, E., Wolfe, G. M., Williamson, C. J., Weinzierl, B., Tilmes, S., Thompson, C. R., Thames, A. B., Schroder, J. C., Saiz-Lopez, A., Rollins, A. W., Roberts, J. M., Price, D., Peischl, J., Nault, B. A., Moller, K. H., Miller, D. O., Meinardi, S., Li, Q. Y., Lamarque, J. F., Kupc, A., Kjaergaard, H. G., Kinnison, D., Jimenez, J. L., Jernigan, C. M., Hornbrook, R. S., Hills, A., Dollner, M., Day, D. A., Cuevas, C. A., Campuzano-Jost, P., Burkholder, J., Bui, T. P., Brune, W. H., Brown, S. S., Brock, C. A., Bourgeois, I., Blake, D. R., Apel, E. C., and Ryerson, T. B.: Global airborne sampling reveals a previously unobserved dimethyl sulfide oxidation mechanism in the marine atmosphere, P. Nat. Acad. Sci. USA, 117, 4505–4510, https://doi.org/10.1073/pnas.1919344117, 2020.
Wang, C., Mattila, J. M., Farmer, D. K., Arata, C., Goldstein, A. H., and Abbatt, J. P. D.: Behavior of isocyanic acid and other nitrogen-containing volatile organic compounds in the indoor environment, Environ. Sci. Tech., 56, 7598–7607, https://doi.org/10.1021/acs.est.1c08182, 2022.
Williams, E. J., Baumann, K., Roberts, J. M., Bertman, S. B., Norton, R. B., Fehsenfeld, F. C., Springston, S. R., Nunnermacker, L. J., Newman, L., Olszyna, K., Meagher, J., Hartsell, B., Edgerton, E., Pearson, J. R., and Rodgers, M. O.: Intercomparison of ground-based NOy measurement techniques, J. Geophys. Res., 103, 22261–22280, https://doi.org/10.1029/98JD00074, 1998.
Williams, J., Roberts, J. M., Bertman, S. B., Stroud, C. A., Fehsenfeld, F. C., Baumann, K., Buhr, M. P., Knapp, K., Murphy, P. C., Nowick, M., and Williams, E. J.: A method for the airborne measurement of PAN, PPN, and MPAN, J. Geophys. Res., 105, 28943–28960, https://doi.org/10.1029/2000jd900373, 2000.
Wolfsie, J. H.: Glycolonitrile toxicity, J. Occup. Med., 2, 588–590, 1960.
Woodrow, J. E., LePage, J. T., Miller, G. C., and Hebert, V. R.: Determination of methyl isocyanate in outdoor residential air near metam-sodium soil fumigations, J. Agr. Food Chem., 62, 8921–8927, https://doi.org/10.1021/jf501696a, 2014.
Zhang, W., and Zhang, H. F.: Secondary ion chemistry mediated by ozone and acidic organic molecules in iodide-adduct chemical ionization mass spectrometry, Anal. Chem., 93, 8595–8602, https://doi.org/10.1021/acs.analchem.1c01486, 2021.
Zhao, G., Quan, D., Zhang, X., Feng, G., Zhou, J., Li, D., Meng, Q., Chang, Q., Yang, X., He, M., and Ma, M.-S.: Glycolonitrile (HOCH2CN) chemistry in star-forming regions, Astrophys. J. Supp. Series, 257, 26, https://doi.org/10.3847/1538-4365/ac17ee, 2021.