the Creative Commons Attribution 4.0 License.
the Creative Commons Attribution 4.0 License.
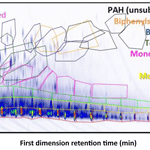
Mapping and quantifying isomer sets of hydrocarbons ( ≥ C12) in diesel exhaust, lubricating oil and diesel fuel samples using GC × GC-ToF-MS
Mohammed S. Alam
Soheil Zeraati-Rezaei
Zhirong Liang
Christopher Stark
Hongming Xu
A. Rob MacKenzie
Airborne particles and vapours, like many other environmental samples including water, soils and sediments, contain complex mixtures of hydrocarbons, often deriving from crude oil either before or after fractionation into fuels, lubricants and feedstocks. Comprehensive 2D gas chromatography time-of-flight mass spectrometry (GC × GC-ToF-MS), offers a very powerful technique that separates and identifies many compounds in complicated hydrocarbon mixtures. However, quantification and identification of individual constituents at high ionization energies would require hundreds of expensive (when available) standards for calibration. Although the precise chemical structure of hydrocarbons does matter for their environmental impact and fate, strong similarities can be expected for compounds having very similar chemical structures and carbon numbers. There is, therefore, a clear benefit in an analytical technique which is specific enough to separate different classes of compounds and to distinguish homologous series while avoiding the need to handle each isomer individually. Varying EI (electron impact) ionization mass spectrometry significantly enhances the identification of individual isomers and homologous compound groups, which we refer to as “isomer sets”. Advances are reported in mapping and quantifying isomer sets of hydrocarbons (≥ C12) in diesel fuel, lubricating oil and diesel exhaust emissions. By using this analysis we report mass closures of ca. 90 and 75 % for diesel fuel and lubricating oil, and identify 85 and 75 % of the total ion current for gas- and particulate-phase diesel exhaust emissions.
- Article
(5632 KB) - Full-text XML
-
Supplement
(1010 KB) - BibTeX
- EndNote
Crude oil contains a highly complex mixture of chemical constituents, mainly hydrocarbons (C4–C55) (Riazi, 2005). There are many reports of crude oil entering the environment through spillage or deliberate release (Gertler et al., 2010). Most crude oil is treated and fractionated in order to produce fuels and lubricants for use in transport and combustion applications, and as feedstocks for the chemical industry (Riazi, 2005). All of these uses have a potential to contaminate the environment. Understanding the fates, pathways and effects of contamination requires chemical analysis and detailed interpretation of resulting data. Much of the chemical complexity of oil derives from the large numbers of straight and branched chain and cyclic hydrocarbon isomers for a given carbon number (Goldstein and Galbally, 2007). Hence, analytical methods are required that can discriminate structurally similar sets of isomers in complex media.
Application of conventional gas chromatographic methods to oils and oil-derived samples was for many years severely limited by the poor separation capability of one-dimensional chromatography due to the near-continuous range of physicochemical properties of hydrocarbons. Thus, typically 90 % of the hydrocarbon content of the sample is present in the unresolved complex mixture (UCM), creating a large hump in the chromatogram (Fraser et al., 1998; Schauer et al., 1999). The advent of two-dimensional gas chromatography, which an provides enhanced separation capability due to the orthogonal separation by two capillary columns of different stationary phases, has transformed the problem by resolving the UCM into many thousands of individual compound peaks. The two columns are connected in series by a modulator which is employed to focus on the primary column eluent (Liu and Phillips, 1991; Phillips and Xu, 1995). Large amounts of data are produced due to the large number of compounds separated. The information which is most useful scientifically in order to compare the main compositional attributes of samples is often more detailed than that provided by bulk total hydrocarbon measurements or fractionation into a few volatility classes but less detailed than specific identification of many compounds. Use of a flame ionization detector has the advantage of allowing generic quantification of any part of the chromatogram in terms of the carbon mass contained within it, but identification of specific chemical constituents with this detector can only be achieved on the basis of retention times which, in a very complex two-dimensional chromatogram and set-up-dependent chromatogram, are laborious to assign objectively. Mass spectrometric detection, especially when employing both low and high ionization energies, adds a third analytical dimension with the ability to overcome the problem of compound identification (Alam et al., 2016a) but has not generally been applied to generic quantification of compound groups within complex samples. In this study, we show that time-of-flight mass spectrometric detection can be used not only to identify and quantify individual chemical constituents within the chromatogram but can also be used to quantify generic groups of compounds.
Motor vehicles are a major source of organic carbon in the atmosphere, and the majority of the fine particulate matter (PM) emitted is carbonaceous, directly emitted as primary organic aerosol (POA) or formed as secondary organic aerosol (SOA) (Jimenez et al., 2009). A substantial fraction of the POA in vehicle emissions has been shown to be semi-volatile under atmospheric conditions (Robinson et al., 2007; May et al., 2013) and is mainly comprised of aliphatic species in the carbon number range between C12 and C35, with effective saturation concentrations (C∗) between 0.1 and 103 µg m−3 (Robinson et al., 2007; Weitkamp et al., 2007). The semi-volatile organic compound (SVOC) composition of lubricating oil has been reported to be dominated by branched, cyclic and straight alkanes (≥ 80 %), with the largest contribution from cycloalkanes (≥ 27 %) (Sakurai et al., 2003; Worton et al., 2014).
Previous research has used a limited range of tracer compounds, or homologous series, for the quantification of emissions, considering representative species that can be distinguished from the bulk of the mass, typically involving analysis of the n-alkanes, polycyclic aromatic hydrocarbons (PAHs), hopanes and steranes (Schauer et al., 1999, 2002), each of which represent only a small fraction of the total mass or number of compounds emitted and might lead to an underestimation of the importance of lubricating oil as a source of SOA (Brandenberger et al., 2005; Fujita et al., 2007). Rogge et al. (1993) investigated the sources of fine organic aerosol from non-catalyst- and catalyst-equipped vehicles using one-dimensional GC-MS but could only resolve 10–15 % of the organics, including n-alkanes and PAHs. Although some studies have utilized soft ionization to analyse diesel fuel at a molecular level (Briker et al., 2001; Eschner et al., 2010; Amirav et al., 2008), very few studies have analysed lubricating oil at a molecular level, which includes the analysis of SVOCs (Worton et al., 2015; Reddy et al., 2012). In order to address the problems of coelution of constituents of the UCM, Worton et al. (2014) and Isaacman et al. (2012) utilized gas chromatography coupled with vacuum ultraviolet ionization mass spectrometry (GC-VUV-MS) to study the constitutional isomers present in lubricating oil and diesel fuel, respectively, and in a standard crude oil from the Gulf of Mexico (Worton et al., 2015). More recently, Goodman-Rendall et al. (2016) used GC-MS with cold electron impact (EI) ionization, resolving detailed molecular components of diesel fuel. Their results showed that the most important factors in determining SOA yields were carbon number, the presence (or absence) of a ring moiety and the degree of substitution; and precise information of branching and degrees of unsaturation was of secondary importance. Dunmore et al. (2015) have shown that diesel-related hydrocarbons are responsible for 60 % of the winter primary hydrocarbon hydroxyl radical reactivity and possibly up to 50 % of the ozone production potential in London. Detailed chemical characterization of diesel emissions would therefore not only resolve factors in determining the contribution to SOA yields but also shed light on specific precursors with large photochemical ozone creation potentials and OH reactivity, as well as the identification of compounds that are harmful for human health and the environment.
As well as identifying individual compounds, using GC × GC allows compounds of similar chemical structure to be classified into distinct groups in ordered chromatograms based on their volatility and their polarity, providing information that aids the identification and assessment of their environmental fate. Dunmore et al. (2015) recently grouped low molecular weight (≤ C12) hydrocarbons in atmospheric samples by carbon number and functionality using GC × GC. They reported the grouping of C6–C13 aliphatics and C2–C4 substituted monoaromatics, combining the area of all the peaks contained within their selected areas.
In our study, two dimensional gas chromatography time-of-flight-mass spectrometry (GC × GC-ToF-MS) (Adahchour et al., 2008; Alam et al., 2013; Alam and Harrison, 2016) was combined with an innovative quantification methodology based on total ion current (TIC) signal response to provide identification and quantification for the compound classes within typical diesel fuel, engine lubricant and engine emissions (gas and particulate phases), providing a near-complete mass closure for diesel fuel and engine lubricant and analyses of diesel engine exhaust composition.
2.1 Sampling
Gas- and particulate-phase diesel exhaust emissions were collected from a light-duty diesel engine. This 2.2 L, 4-cylinder, in-line compression ignition engine was equipped with a common rail direct injection system and a variable nozzle turbine (VNT) turbocharger. Samples were collected with no diesel oxidation catalyst (DOC) and diesel particulate filter (DPF). The diesel engine emissions were diluted (1 : 50) with cleaned compressed air using an in-house exhaust dilution system. Samples were collected at steady-state engine operating conditions at a low engine load of 3.0 bar mean effective pressure (BMEP) and a speed of 1800 revolutions per minute (RPM). The temperature at the sampling point was 25 ± 5 ∘C and samples were collected for 30 min at a flow rate of 1.8 L min−1. Adsorption tubes were used to collect gas-phase constituents directly from the diluted diesel engine exhaust, downstream of a polypropylene-backed PTFE 47 mm filter (Whatman, Maidstone, UK), which was used to collect constituents in the particulate phase. Further details of the engine exhaust sampling system are given elsewhere (Alam et al., 2016b).
Diesel fuel, engine lubricating oil and gas-and-particulate diesel exhaust emission samples were analysed using GC × GC-ToF-MS. Briefly, 1 µL of diesel fuel (EN 590-ultra low sulfur diesel, Shell, UK) was diluted (1 : 1000) in dichloromethane (DCM) and injected onto a stainless steel thermal adsorption tube, packed with 1 cm quartz wool, 300 mg Carbograph 2TD 40/60 (Markes International, Llantrisant, UK), for analysis on the thermal desorber (TD) coupled to the GC × GC-ToF-MS. The EN 590 ultra-low sulfur diesel fuel is representative of the standardized ultra-low sulfur content fuel (< 10 mg kg−1 or ppm) that is widely used in the UK and Europe (European Parliament and the Council of the European Union, 2009). 1 µL of engine lubricant (fully synthetic, 5W30, Castrol, UK) was diluted (1 : 1000) in DCM and directly injected into the gas chromatographic column, as the high molecular weight constituents found in the lubricating oils would not efficiently desorb into the GC column from the adsorption tubes.
2.2 Instrumentation
Adsorption tubes were desorbed using TD (Unity 2, Markes International, Llantrisant, UK) and samples were subsequently analysed using a gas chromatograph (GC, 7890B, Agilent Technologies, Wilmington, USA) equipped with a Zoex ZX2 cryogenic modulator (Houston, USA). The primary column (first separation dimension) was equipped with a SGE DBX5, non-polar capillary column (30 m, 0.25 mm ID, 0.25 µm – 5 % phenyl polysilphenylene-siloxane). The secondary, more polar column (second separation dimension) was equipped with a SGE DBX50 (4.0 m, 0.1 mm ID, 0.1 µm – 50 % phenyl polysilphenylene-siloxane), situated in a secondary internal oven. The GC × GC was interfaced with a BenchTOF-Select time-of-flight mass spectrometer (ToF-MS, Markes International, Llantrisant, UK), with a scan speed of 50 Hz and a mass resolution of > 1200 full width at half maximum (fwhm) at 70 eV and > 800 fwhm at 14 eV over 100–1000 m∕z. The mass/charge range was 30 to 525 m∕z, and quantification was conducted on a nominal unit mass resolution. Electron impact ionization energies on this ToF-MS can be tuned between 10 and 70 eV, the former retaining the molecular ion and the latter causing extensive fragmentation, but it allows comparison with standard library spectra (Alam et al., 2016a). Data were processed by using GC Image v2.5 (Zoex Corporation, Houston, USA).
2.3 Standards and chromatography methodology
Nine deuterated internal standards, namely dodecane-d26, pentadecane-d32, eicosane-d42, pentacosane-d52, triacontane-d62, biphenyl-d10, n-butylbenzene-d14, n-nonylbenzene-2,3,4,5,6-d5 (Chiron AS, Norway) and p-terphenyl-d14 (Sigma Aldrich, UK) were utilised for quantification. Natural standards included 24 n-alkanes (C11–C34), phytane and pristane (Sigma Aldrich, UK), 16 n-alkylcyclohexanes (C11–C25 and C26), 5 n-alkylbenzenes (C4, C6, C8, C10 and C12), cis- and trans-decalin, tetralin, 4 alkyltetralins (methyl-, di-, tri- and tetra-), 4 n-alkyl naphthalenes (C1, C2, C4 and C6) (Chrion AS, Norway) and 16 USEPA polycyclic aromatic hydrocarbons (Thames Restek UK Ltd). These standards were chosen in order to cover as much of the overall chromatogram as possible. The chromatography methodology (GC oven temperatures, temperature ramp rates, etc.) of the analysis of the adsorption tubes, lubricating oil and gas/particulate-phase samples is discussed in Sect. S1 in the Supplement.
2.4 Grouping of chromatographically resolved compounds
Structurally similar compounds possess similar physicochemical properties. This facilitates identification when separating a mixture according to these physical and chemical properties. Diesel fuels, diesel emissions and lubricating oils have been shown to consist of a limited number of compound classes, but an enormous number of individual components within a class.
In this study we use GC × GC coupled to variable ionization ToF-MS to map and quantify isomer sets previously unresolved in the UCM. Conventional electron ionization at 70 eV imparts a large amount of excess energy causing extensive fragmentation, with a tendency to generate similar mass spectra. For example, the isomeric alkanes all exhibit the same m∕z 43, 57, 71, 85, 99 patterns, thus obscuring the match with the NIST library and making identification from the mass spectrum very difficult. To address this issue, a lower ionization energy (10–14 eV) was also employed in our study so that the organic compounds are ionized with minimal excess internal energy and thus less fragmentation, hence retaining the distinct identity of the molecule with a much larger fraction of the molecular ion (Alam et al., 2016a). Running samples on the GC × GC with both low and high ionization energy mass spectrometry results in a wealth of data for identification of compounds; where 14 and 70 eV mass spectra can be compared for a given species owing to the identical retention times of the repeat runs. At low ionization energy the molecular ion is enhanced, retaining some fragmentation at the same time, while at high ionization energy the mass fragmentation patterns of a species can be compared directly to mass spectral libraries. This allows easier identification of unknown compounds. Low EI spectra therefore give qualitative information, while high EI mass spectra allow for quantitative analyses to be performed.
Our recent work exploited soft ionization (14 eV) to identify a large number of isomers, demonstrating the ability to separate and identify individual alkanes (normal, branched and cyclic) with specific carbon numbers, based on their volatility and polarity (Alam et al., 2016a). In this study we expand our previous qualitative analysis and separate the alkane series (as well as other homologous series) into isomer sets containing the same carbon number. Individual alkanes that were identified as having different molecular ions (i.e. different carbon number) to the n-alkane within the area of the chromatogram were included in their appropriate adjacent (usually n ± 1) area; for example, some dimethyl isomers can be shifted by ∼ 100 delta Kovats (∼ 1 carbon number), whereas trimethyl- and tetramethyl isomers have been reported to be shifted by ∼ 150 and ∼ 200 delta-Kovats. This has been completed for all the homologous series reported in this study. The grouping of the alkanes according to their respective carbon numbers is shown in Fig. 1, where the least polar compounds (fast eluting peaks in the second dimension) are the alkanes, increasing in carbon number as the retention time in the first dimension increases.
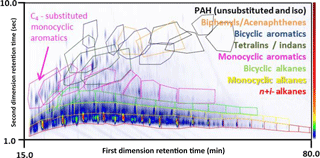
Figure 1A contour plot (chromatogram with 70 eV ionization mass spectrometry) of diesel fuel separation. Peak height (intensity) increases with the warmth (blue to red) of the colour scale. Each region fenced by a coloured polygon marks out the two-dimensional chromatogram space in which isomers of a particular compound type are found with a particular carbon number (e.g. C4–substituted monocyclic aromatics).
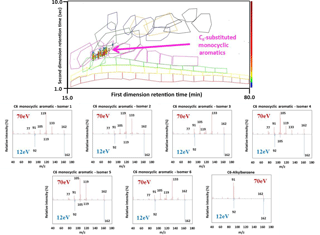
Figure 2A contour plot (chromatogram with 12 eV ionization mass spectrometry) displaying C6-substituted monocyclic aromatics identified by the CLIC expression. All C6-substituted monocyclic aromatics are located within the pink polygon displayed. 70 eV (red peaks) and 12 eV (blue peaks) mass spectra corresponding to the peaks identified by the CLIC expressions in the SIC is shown for six different C6-substituted monocyclic aromatics isomers.
This methodology was expanded to more polar homologous series including monocyclic alkanes, bicyclic alkanes, tricyclic alkanes, tetralins/indanes, monocyclic aromatics, bicyclic aromatics and alkyl-biphenyls. Like the alkanes, a significant problem in creating the boundaries of groups is the overlapping of one carbon number group into another. Identifying each individual compound in this case (as with the alkanes above) would be resource and time intensive and so carefully constructed Computer Language for Identification of Chemicals (CLIC) qualifiers were created and utilised in order to match peaks and their mass fragmentation patterns. A CLIC qualifier is an expression in a computer language that allows users of chromatographic software to build rules for selecting and filtering peaks using retention times and mass fragmentation patterns (Reichenbach et al., 2005). This was exploited to identify specific compounds belonging to a compound class and a polygon selection tool within the GC Image software was drawn around this section of the chromatogram (coloured polygons shown in Fig. 1). Any overlap in the graphics was accounted for by forcing peaks to belong to one compound class over another via strict mass fragmentation and molecular ion selection tools. Examples of CLIC expressions utilised for identifying compound classes are included in the Supplement (Sect. S2). An example of a selected ion chromatogram with a specific CLIC expression is shown in Fig. 2, for C6-substituted monocyclic aromatics, with their corresponding 70 and 12 eV mass spectra. The characteristic 70 eV mass fragments at m∕z 92, 105, 119, 133 signify cleavage of the C-C bond next to the benzene ring. The 12 eV mass spectra, however, produce poor characteristic fragment ions, but a prominent molecular ion (162) and m∕z 92 signifying the overall mass of the molecule and the benzene ring (), respectively. In effect, the polygons mark out sets of isomeric compounds with the same empirical formula and shared structural elements; the sets appear to intersect each other in the two-dimensional chromatogram space, but compounds in the intersecting regions are assigned uniquely to a class using a third mass spectrometric data dimension (i.e. mass fragmentation patterns). The resulting isomer sets are more chemically and environmentally meaningful than the raw polarity/volatility assignment from the chromatography. This approach was completed independently for diesel fuel, lubricating oil and gas/particulate-phase exhaust emissions to ensure the applicability of polygon boundaries and reproducibility of retention times and mass fragments. Results indicated that isomers within the constructed polygon boundaries possessed identical retention times and interpretable mass spectra for all differing samples. Retention times were reproducible in both chromatographic dimensions in separate runs. The isomer sets (polygons) can be linked together in a large template that can also be linked to the internal standards. This allows easy alignment of the template (all isomer sets) in the event of slight shifts in retention times due to column changes or instrumental maintenance.
2.5 Quantification of compounds with no authentic standards
The authentic standard mixture contains 74 standard compounds, including 9 internal standards (see Sect. 2.3). These standards were chosen in order to cover as much of the overall chromatogram as possible and are used for obtaining a calibration for quantifying groups of isomers with the same molecular ion and functionality. For example, the response for C11 (undecane, m∕z 156) was used to quantify all C11 alkane isomers which were positively identified in the analysed samples (and have the same molecular mass and retention times in all sample runs). Using retention times for C11 alkane isomers as well as mass spectra, only those C11 isomer peaks that were observed using the SIC for m∕z 156 (or the respective CLIC expressions) were selected. Isomer sets were comprehensively identified in the analysed samples using mass fragmentation at 70 eV and molecular masses at lower ionization energies (10–15 eV). The total ion current within each polygon was integrated and the isomer set abundance was estimated using the response ratio of the closest structurally related deuterated standard to the corresponding compound class natural standard with the same carbon number (usually within the polygon). This methodology has an uncertainty of approximately 24 % and is discussed in detail in the Supplement (Sect. S3).
Table 1Hydrocarbons identified in diesel fuel, lubricating oil and diesel exhaust emissions (gas and particulate phases) with their respective m∕z fragment ions and percentage mass contributions.
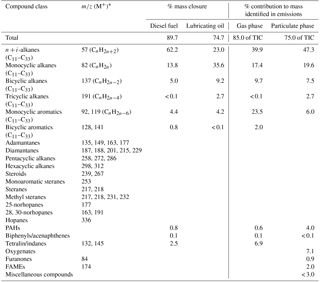
∗ m∕z ratios presented here are the main mass fragments present in the low ionization energy mass spectra. CLIC expressions and 70 eV mass spectra use more m∕z fragments which were also used for qualification and quantification.
3.1 Analysis of diesel fuel
The chromatography of the diesel fuel analysed by TD-GC × GC-ToF-MS is shown in Fig. 1. Compounds identified within the diesel fuel included n-alkanes, branched alkanes (mono-, di-, tri-, tetra- and penta-methyl), n-alkyl cycloalkanes, branched monocyclic alkanes, C1–C12 substituted bicyclic alkanes, C1–C4 substituted tetralins and indanes, C3–C12 substituted monocyclic aromatics, C1–C3 substituted biphenyls/acenaphthenes, C1–C4 substituted bicyclic aromatics, C1–C2 substituted fluorenes (FLU), C1–C2 substituted phenanthrene/anthracenes (PHE/ANT) and unsubstituted PAHs. Representative mass spectra at 12 and 70 eV ionization are presented in the Supplement (Sect. S4). These compounds accounted for 93 % of the total response (excluding the siloxanes which derive from contaminants, i.e. column bleed) and was equivalent to 90 % of the mass injected. Therefore, out of the 8026 (±24) ng that was injected into the GC × GC (mass calculated by weighing and diluting a known volume of diesel fuel, while the uncertainty represents the combined uncertainties of the processes involved in this estimation) a mass of approximately 7200 (±1728) ng was accounted for. We suspect that a significant amount of the mass that was unaccounted may be < C10 and/or any unresolved peaks that we were unable to measure and/or identify using our technique. The percentage contribution of each compound class identified to the total mass accounted for is shown in Table 1.
Our results indicate that the majority of the diesel fuel consists of aliphatic compounds, with a low aromatic content (∼ 10 %). Very few published studies exist elucidating the contribution of different constituents in diesel fuel (Isaacman et al., 2012; Welthagen et al., 2007; Gentner et al., 2012). Most studies focus on the characterization of specific compounds within diesel fuels such as nitrogen-containing species (Wang et al., 2004) or cyclic compounds (Edam et al., 2005) to identify strengths and weaknesses in analytical techniques (Frysinger and Gaines, 1999). Recently, VUV ionization at 10–10.5 eV has been exploited to elucidate some of the structures within diesel fuel, by separating the components using GC (Isaacman et al., 2012). The authors report their observed mass of diesel fuel as 73 % aliphatic and 27 % aromatic, which is broadly consistent with the results of this study. Up to 11 % of the observed mass fraction of diesel fuel was attributed to bicyclic alkanes, a factor of 2 larger than observed in this study. Their observed mass fractions of cycloalkanes and benzene, however, are in excellent agreement. The contributions of branched alkanes (i-alkanes) and linear n-alkanes to the total mass of the alkanes were 39.1 and 23.1 %. A significant proportion of the total mass observed was attributed to alkanes (62 %), a factor of 1.5 larger than reported by Isaacman et al. (2012). However, the differences observed between diesel fuel analysed in this study and that reported by Isaacman et al. (2012) are attributable to different fuel formulations and/or fuel source, as opposed to analytical methods. Although not shown here, a significant number of alkane isomers were identified for each carbon number using soft ionization mass spectrometry, accounting for a total of ∼ 200 alkanes across the C11–C30 range. The ratio of i-alkanes to n-alkanes sharply decreases after C25, indicating a reduced amount of mass represented by branched isomers present in diesel fuel for > C25 alkanes, which could be related to the formulation process, or reflect the composition of the feedstock.
3.2 Analysis of diesel engine emissions (gas phase)
A GC × GC contour plot of the gas-phase diesel exhaust emissions is shown in the Supplement (Fig. S5). The observed chromatogram for the gas-phase emissions looked extremely similar to that of the diesel fuel chromatogram (Fig. 1), suggesting that the majority of compounds found in the gas-phase emissions are of diesel fuel origin. All of the compounds found in the diesel fuel were observed in the gas-phase emissions, albeit with a reduced number of i-alkanes > C20, which may signify efficient combustion of these high molecular weight compounds, or partitioning into the particulate phase. The measured constituents of the gas-phase diesel exhaust emissions are shown in Table 1. Approximately 15 % of the total ion current (response, excluding siloxanes) was unaccounted for. Table 1 illustrates the percentage mass of each compound class identified, in the 85 % of the response that was accounted for. As the total mass of the gas-phase sample is unknown, a mass for the remaining 15 % of the total response cannot be estimated, as the individual components that are unidentified will have different responses per unit mass. For example, 23.5 % of the mass identified was attributed to C4–C12 alkyl-substituted monocyclic aromatics and accounted for 9.7 % of the total ion current response; and 10.0 % of the mass identified was bicyclic alkanes, representing 9.2 % of the response.
Although the diesel fuel constituents present in the gas-phase exhaust emissions broadly were compositionally consistent with the fuel, there were significant differences observed in their relative amounts. Of the total mass identified in the gas-phase emissions, n-alkanes and i-alkanes represented 9.8 and 30.1 %. These are factors of 2.4 and 1.3 lower than that for diesel fuel, respectively, which may be due to preferred combustion of these compounds (Burcat et al., 2012). Enhancements in monocyclic aromatics, monocyclic alkanes, bicyclic alkanes and bicyclic aromatics were observed in the emissions, possibly due to them being intermediate species formed during the combustion of larger molecules (Gentner et al., 2013). They are unlikely to be a contribution from lubricating oil as very little mass was attributed to compounds with < C18 (see Sect. 3.3). A very limited number of oxygenates were also identified (e.g. ketones, m∕z 58, 72; carboxylic acids, m∕z 60), most probably combustion products of diesel fuel, but they represent a very small fraction of the total measured gas-phase emissions (< 1 %). However, carboxylic acids are difficult to detect using GC-MS without prior derivatization and may therefore be underestimated. Gentner et al. (2013) suggest that compounds such as alkenes, aromatics and oxygenates comprise ∼ 30 % of the total measured gas-phase emissions, in agreement with this study; however, they suggest that these products are unlikely to contribute to primary organic aerosol (POA). We observe these aromatic compounds in the particulate phase also, which indicate a contribution.
3.3 Analysis of lubricating oil
The isomer sets (polygons) identified in the lubricating oil chromatogram are shown in Fig. 3a. Molecular ions present in the mass spectra enabled the grouping of isomers by carbon number, while the presence of the characteristic mass fragments, presented in Table 1, were used to confirm the identity of the type of hydrocarbon. The representative mass spectra for compounds presented in Table 1, for the lubricating oil is shown in the Supplement, Sect. S6. Polygons were drawn around groups of compounds that possessed the same molecular ion for a given compound class; see Fig. 3a and b. The lubricating oil was analysed using two independent temperature ramps of the GC × GC (methodologies outlined above): one to achieve the best possible comprehensive separation of compounds in the oil (Fig. 3a) and the other using methodologies developed for analysis of the particulate-phase components of engine exhaust (Fig. 3b) to ascertain where the compounds identified in the oil are present in the particulate-phase emissions filter. Figure 3b also illustrates the positioning of the SVOC measured in the gas phase, which are observed in the particulate-phase filter as well as the positioning for the PAHs. The grouping template that is illustrated in Fig. 1 covers the SVOC range indicated in Fig. 3b.
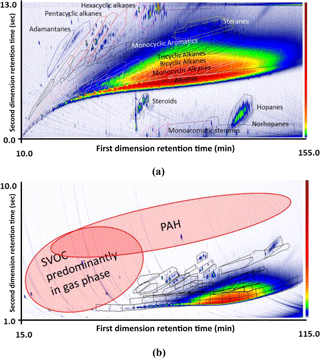
Figure 3A chromatogram (with 70 eV ionization mass spectrometry) of lubricating oil (5W30) (a) with labelled compound classes, using a methodology specific for characterizing the composition of lubricating oil, (b) using methodology developed for characterizing particulate-phase emissions from diesel engine exhaust. Polygons drawn around sections of the chromatograms indicate compounds with different molecular masses within compound classes.
Using the signature mass fragment ions (Table 1) together with the calculated molecular mass, specific compounds with the same carbon number were isolated; see Fig. S7-A and S7-B. For example, selecting the ion fragment m/z 92 and 119 for monocyclic aromatics gives rise to the selected ion chromatogram illustrated in Fig. S7-A. This can be achieved using 70 eV mass spectrometry identifying a homologous series across a large carbon number range. However, selecting the molecular mass for a specific carbon number allows the identification of all isomer sets in a region of the chromatogram with that specific molecular mass, as shown in Fig. S7-B for C22 monocyclic aromatics (m/z 302). A mass of 8511 (±255) ng of lubricating oil was injected into the GC × GC, of which 6356 (±1525) ng was quantified. This methodology was used to identify and quantify the following homologous series: C16–C33 straight and branched chain alkanes, C16–C33 monocyclic alkanes, C17–C33 bicyclic alkanes, C17–C33 tricyclic alkanes and C16–C33 monocyclic aromatics. These compound groups represented approximately 91 % of the total ion current (excluding siloxanes) and 75 % of the mass fraction. Adamantanes, diamantanes, pentacyclic and hexacyclic alkanes, steroids, steranes and hopanes represented 5 % of the total ion current, while the remaining 4 % remained unidentified. These compounds were not quantifiable using this methodology, as there were no standards available that corresponded to these sections of the chromatography and could not be estimated as they are not present in a homologous series. However, from previous literature, these compound classes are thought to represent a small fraction of the mass (Worton et al., 2015). Furthermore, we have not taken into account any non-organic/hydrocarbon species but according to these data, the fraction of any non-HCO material is likely to be small.
Worton et al. (2015) exploited VUV photoionization mass spectrometry to comprehensively characterize hydrocarbons in a standard reference crude oil sample. They reported a total mass closure of 68 ± 22 %, comprised of linear and branched alkanes (19 %), 1–6 ring cycloalkanes (37 %), monoaromatics (6.8 %) and PAHs (4.7 %). The mass fractions observed for linear and branched alkanes in this study were 11 and 12 %, which is in excellent agreement. There is also excellent agreement with the mass attributed to bicyclic (2-ring) and tricyclic (3-ring) alkanes. However, for monocyclic alkanes the results presented here are a factor of 2 larger than Worton et al. (2015) and 2.5 larger than Reddy et al. (2012). Both previous studies analysed similar crude oil samples associated with the Deepwater Horizon disaster (McNutt et al., 2012) and would be expected to differ appreciably from a lubricating oil. Furthermore, no PAHs were observed in the lubricating oil in this study, in agreement with Zielinska et al. (2004) but in contrast to Worton et al. (2015). We attribute this difference to the varying crude oil origins and formulation processes involved in the production of synthetic oil.
Previous work from this group identified a large number of isomeric species in base oil using 14 eV EI ionization energy mass spectrometry (Alam et al., 2016a). Although we were able to identify a large number of compounds, there still existed a small amount of fragmentation at 14 eV, particularly for alkyl-methyl-, alkyl-dimethyl- and alkyl-trimethyl-cyclohexanes. In this study the fragmentation was significantly reduced for these compounds at 12 eV (i.e. relative intensities of m∕z 97, 111, 125 reduced by > 50 %) and completely eradicated (relative intensities of mass fragments reduced by > 95 %) at 10 eV, leaving the m∕z 82 ion (for monocyclic alkanes) and the molecular ion. This demonstrates the significant differences observed in fragmentation over small changes in lower ionization EI energies and may also account for slight discrepancies between studies (Worton et al., 2015; Isaacman et al., 2012; Alam and Harrison, 2016). Utilizing these differences in fragmentation from using low ionization energies (10–15 eV) may provide more information in regards to the structure of many isomeric compounds.
3.4 Analysis of diesel engine emissions (particulate phase)
90 % of the total ion current of the particulate-phase filter was identified and attributed to a wide range of classes. Of the total mass identified, 47 (±11) % was straight and branched chain alkanes, 20 (±4.8) % monocyclic alkanes, 7.5 (±1.8) % bicyclic alkanes, < 3 (±0.7) % tricyclic alkanes, 6 (±1.4) % monocyclic aromatics, 7 (±1.7) % oxygenates, < 1 (±0.2) % furanones, 4 (±1.0) % PAHs and 2 (±0.5) % fatty acid methyl esters (FAMES). Figure 4 illustrates the percentage mass contribution of homologous series (including isomers) identified as a function of carbon number. Peak concentrations of alkanes (cyclic and straight/branched chain) were observed between C24–C27 consistent with the lubricating oil, while a small peak in concentration was also observed in the C15–C20 range, consistent with the fuel and gas-phase emissions. Oxygenated compounds were found to be present in the C11–C22 range, suggesting that these compounds are combustion products. The concentration of monocyclic aromatics was steady throughout the carbon number distribution (C15–C32), with a small peak at C25–C27. The presence of PAHs in the particulate-phase suggests their formation via diesel fuel combustion or unburnt fuel, owing to their absence in the lubricating oil. There are numerous studies reporting the absence of PAHs in unused lubricating oil and presence in used oil, which suggests the absorption of an exhaust blow-by containing fuel-combustion-associated PAHs (Fujita et al., 2006). FAMES were identified by their characteristic fragmentation at 70 eV EI ionization and with their characteristic ion (m∕z 174) at low EI ionization (12 eV).
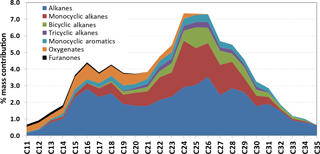
Figure 4Percentage mass contribution of the compounds identified in homologous series as a function of carbon number in diesel exhaust particles.
There have been few studies investigating the contribution of lubricating oil and fuel to the emitted diesel POA, suggesting 20 to 80 % influence from lubricating oil (Worton et al., 2014; Brandenberger et al., 2005; Kleeman et al., 2008; Sonntag et al., 2012). Most recently, it has been suggested that ≥ 80 % of the SVOC composition is dominated by branched cycloalkanes with one or more rings and one or more branched alkyl side chain (Worton et al., 2014). This is significantly larger than that reported in this study (≥ 30 %), where the majority of the emissions are dominated by straight and branched chain alkanes (47 %) over a volatility range that also suggests a significant contribution from the diesel fuel (C11–C20; see Fig. 4). The diesel fuel and lubricating oil contained respectively 62 and 47.5 % straight and branched chain alkanes (summed), suggesting a larger possible contribution of diesel fuel to the vapour-phase engine emissions (which is dominated by straight and branched chain alkanes). The contribution of unburned lubricating oil, however, most likely dominates the SVOC emissions in the particulate phase, as shown in Fig. 4.
The SVOC contents in diesel fuel, 5W30 synthetic lubricating oil and diesel exhaust emissions (both in the gas and particulate phases) were characterized using TD-GC × GC-ToF-MS. By exploiting the mass spectrometric fingerprint of eluting compounds in highly structured and ordered chromatograms, a method has been constructed that quantifies the contributions of “isomer sets” (i.e. structural isomers in specific compound classes) to the overall composition of a sample. We found that the ion current for identified homologous series exhibited very similar responses, illustrating that quantitative calibrations derived from the n-alkane series could be used to estimate the concentrations of isomeric aliphatic compounds with similar molecular weight. Using this methodology together with a range of standards and aggregating compound classes of similar functionality together (n-alkanes, branched alkanes, etc.), we present a comprehensive characterization of diesel fuel, lubricating oil and diesel exhaust emissions.
Furthermore, combining conventional 70 eV EI ionization mass spectrometry with lower ionization energy (10–14 eV), allowed the identification of constitutional isomers of the same molecular weight and compound class, enabling a clear distinction between carbon number and functionality. By utilizing this innovative method, a number of findings were achieved. (1) A mass closure accounted for ca. 90 and 75 % for the analyses of diesel fuel and lubricating oil. (2) Acyclic and monocyclic alkanes were found to be predominant in both the diesel fuel and synthetic lubricating oil (76 and 68 %). (3) Diesel exhaust emissions in the gas phase had an extremely similar composition to diesel fuel. (4) Diesel exhaust emissions in the particulate phase had an extremely similar composition to lubricating oil. (5) The presence of combustion products of diesel fuel (e.g. aromatics and oxygenates) in the particulate phase indicates a contribution to POA.
Diesel exhaust hydrocarbons are a significant precursor of secondary organic aerosol (Dunmore et al., 2015; Gentner et al., 2012). Diesel fuel and lubricant, contributors to diesel exhaust, contain large numbers of isomers. Separation into isomer sets improves our understanding of the fates of these oil-derived materials in the environment (Lim and Ziemann, 2009; Kroll and Seinfeld, 2008). By utilizing GC × GC-ToF-MS with soft ionization, we enable the identification of the composition of the UCM, characterizing the chemical composition by carbon number and compound class, and the possibility of branching structural information. Along with a grouping methodology using CLIC expressions and unique compound fragmentation patterns, we demonstrate the reliable quantitative integration of structural isomers. These methods exploit the improved resolution and isomer separation capabilities of the advanced instrumentation and have potential applications to the observations of petroleum degradation, and SOA formation and evolution. This method is ideal for investigating fossil fuel sources (e.g. lubricating oil, motor vehicles and fuel) and can be extended to atmospheric measurements where many oxygenates exist. The analysis of atmospheric particulate-phase samples (not using thermal desorption) may benefit from exploring different extraction solvents (e.g. methanol or a hexane/methanol mix) to allow the larger than expected proportion of oxygenated species to be efficiently extracted. Although the presence of oxygenated species adds chromatographic complexity, as co-elution can be a limitation, using carefully constructed CLIC expressions and mass fragmentation patterns, various oxygenates can be identified (e.g. 2-ketones, m∕z 58; 3-ketones, m∕z 72; and carboxylic acids, m∕z 60), making this technique applicable in any scientific field that routinely characterizes complex hydrocarbon mixtures. Although not explored here, this technique of low ionization energy mass spectrometry may benefit from reducing the temperature of the ionization source, as demonstrated by Isaacman et al. (2012), who retained a larger fraction of the molecular parent ion when reducing the ion source temperature of their VUV photoionization technique. This would particularly aid the identification of atmospheric samples, which would contain more oxygenated compounds.
Data presented and analysed in this paper are available from the corresponding author on request.
The supplement related to this article is available online at: https://doi.org/10.5194/amt-11-3047-2018-supplement.
MSA prepared the manuscript with contributions from ARM and RMH; RMH, MSA and SZR designed the engine experiments; SZR, MSA and ZL carried out the engine experiments; MSA and CS developed the GC × GC methodology and completed subsequent analyses. HX overlooked the engine facility and RMH oversaw the entire project.
The authors declare that they have no conflict of interest.
The authors would like to thank Laura McGregor and Nick Bukowski for valuable
discussions on aspects of GC × GC-ToF-MS and assistance in CLIC
qualifiers. Financial support from the European Research Council
(ERC-2012-AdG, proposal no. 320821) for the FASTER project is gratefully
acknowledged. The assistance of Yasser Al-Qahtani in collecting samples from the
engine facility is also acknowledged.
Edited by: Pierre Herckes
Reviewed by: two anonymous referees
Adahchour, M., Beens, J., and Brinkman, U. A.: Recent developments in the application of comprehensive two-dimensional gas chromatography, J. Chromatogr. A, 1186, 67–108, 2008.
Alam, M. S. and Harrison, R. M.: Recent advances in the application of 2-dimensional gas chromatography with soft and hard ionisation time-of-flight mass spectrometry in environmental analysis, Chemical Science, 7, 3968-3977, 2016.
Alam, M. S., West, C. E., Scarlett, A. G., Rowland, S. J., and Harrison, R. M.: Application of 2D-GCMS reveals many industrial chemicals in airborne particulate matter, Atmos. Environ., 65, 101–111, 2013.
Alam, M. S., Stark, C., and Harrison, R. M.: Using Variable Ionization Energy Time-of-Flight Mass Spectrometry with Comprehensive GC × GC to Identify Isomeric Species, Anal. Chem., 88, 4211–4220, 2016a.
Alam, M. S., Zeraati-Rezaei, S., Stark, C. P., Liang, Z., Xu, H., and Harrison, R. M.: The characterisation of diesel exhaust particles – composition, size distribution and partitioning, Faraday Discuss., 189, 69–84, 2016b.
Amirav, A., Gordin, A., Poliak, M., and Fialkov, A. B.: Gas chromatography-mass spectrometry with supersonic molecular beams, J. Mass Spectrom., 43, 141–163, 2008.
Brandenberger, S., Mohr, M., Grob, K., and Neukom, H. P.: Contribution of unburned lubricating oil and diesel fuel to particulate emission from passenger cars, Atmos. Environ., 39, 6985–6994, 2005.
Briker, Y., Ring, Z., Iacchelli, A., McLean, N., Rahimi, P. M., and Fairbridge, C.: Diesel fuel analysis by GC-FIMS: Aromatics, n-paraffins, and isoparaffins, Energy and Fuels, 15, 23–37, 2001.
Burcat, A., Dixon-Lewis, G., Frenklach, M., Hanson, R. K., Salimian, S., Troe, J., Warnatz, J., and Zellner, R.: Combustion chemistry, edited by: Gardiner, W. J., Springer-Verlag New York Inc., New York, NY, USA, 2012.
Dunmore, R. E., Hopkins, J. R., Lidster, R. T., Lee, J. D., Evans, M. J., Rickard, A. R., Lewis, A. C., and Hamilton, J. F.: Diesel-related hydrocarbons can dominate gas phase reactive carbon in megacities, Atmos. Chem. Phys., 15, 9983–9996, https://doi.org/10.5194/acp-15-9983-2015, 2015.
Edam, R., Blomberg, J., Janssen, H.-G., and Schoenmakers, P.: Comprehensive multi-dimensional chromatographic studies on the separation of saturated hydrocarbon ring structures in petrochemical samples, J. Chromatogr. A, 1086, 12–20, 2005.
Eschner, M. S., Welthagen, W., Groger, T. M., Gonin, M., Fuhrer, K., and Zimmermann, R.: Comprehensive multidimensional separation methods by hyphenation of single-photon ionization time-of-flight mass spectrometry (SPI-TOF-MS) with GC and GC × GC, Analytical Bioanalytical Chemistry, 398, 1435–1445, 2010.
European Parliament and Council of the European Union: Directive 2009/30/EC of the European parliament and of the council of 23 April 2009, Official Journal of the European Union, L140, 88–113, 2009.
Fraser, M. P., Cass, G. R., and Simoneit, B. R.: Gas-phase and particle-phase organic compounds emitted from motor vehicle traffic in a Los Angeles roadway tunnel, Environ. Sci. Technol., 32, 2051–2060, 1998.
Frysinger, G. S. and Gaines, R. B.: Comprehensive Two-Dimensional Gas Chromatography with Mass Spectrometric Detection (GC × GC/MS) Applied to the Analysis of Petroleum, J. High Res. Chromatog., 22, 251–255, 1999.
Fujita, E. M., Campbell, D. E., and Zielinska, B.: Chemical analsis of lubricating oil samples from a study to characterize exhaust emissions from light-duty gasoline vehicles in the Kansas City Metropolitan area, Final Report, Desert Research Institute, Reno, Nevada, USA, 2006.
Fujita, E. M., Zielinska, B., Campbell, D. E., Arnott, W. P., Sagebiel, J. C., Mazzoleni, L., Chow, J. C., Gabele, P. A., Crews, W., and Snow, R.: Variations in speciated emissions from spark-ignition and compression-ignition motor vehicles in California's south coast air basin, J. Air Waste Manage., 57, 705–720, 2007.
Gentner, D. R., Isaacman, G., Worton, D. R., Chan, A. W. H., Dallmann, T. R., Davis, L., Liu, S., Day, D. A., Russell, L. M., Wilson, K. R., Weber, R., Guha, A., Harley, R. A., and Goldstein, A. H.: Elucidating secondary organic aerosol from diesel and gasoline vehicles through detailed characterization of organic carbon emissions, P. Natl. Acad. Sci. USA, 109, 18318–18323, 2012.
Gentner, D. R., Worton, D. R., Isaacman, G., Davis, L. C., Dallmann, T. R., Wood, E. C., Herndon, S. C., Goldstein, A. H., and Harley, R. A.: Chemical composition of gas-phase organic carbon emissions from motor vehicles and implications for ozone production, Environ. Sci. Technol., 47, 11837–11848, 2013.
Gertler, C., Yakimov, M. M., Malpass, M. C., and Golyshin, P. N.: Shipping-related accidental and deliberate release into the environment, Handbook of Hydrocarbon and Lipid Microbiology, Springer, Berlin, Heidelberg, Germany, 243–256, 2010.
Goldstein, A. H. and Galbally, I. E.: Known and unexplored organic constituents in the Earth's atmomsphere, Environ. Sci. Technol., 41, 1514–1521, 2007.
Goodman-Rendall, K. A., Zhuang, Y. R., Amirav, A., and Chan, A. W. H.: Resolving detailed molecular structures in complex organic mixtures and modeling their secondary organic aerosol formation, Atmos. Environ., 128, 276–285, 2016.
Isaacman, G., Wilson, K. R., Chan, A. W. H., Worton, D. R., Kimmel, J. R., Nah, T., Hohaus, T., Gonin, M., Kroll, J. H., Worsnop, D. R., and Goldstein, A. H.: Improved resolution of hydrocarbon structures and constitutional isomers in complex mixtures using gas chromatography-vacuum ultraviolet-mass spectrometry, Anal. Chem., 84, 2335–2342, 2012.
Jimenez, J. L., Canagaratna, M. R., Donahue, N. M., Prevot, A. S. H., Zhang, Q., Kroll, J. H., DeCarlo, P. F., Allan, J. D., Coe, H., Ng, N. L., Aiken, A. C., Docherty, K. S., Ulbrich, I. M., Grieshop, A. P., Robinson, A. L., Duplissy, J., Smith, J. D., Wilson, K. R., Lanz, V. A., Hueglin, C., Sun, Y. L., Tian, J., Laaksonen, A., Raatikainen, T., Rautiainen, J., Vaattovaara, P., Ehn, M., Kulmala, M., Tomlinson, J. M., Collins, D. R., Cubison, M. J., Dunlea, E. J., Huffman, J. A., Onasch, T. B., Alfarra, M. R., Williams, P. I., Bower, K., Kondo, Y., Schneider, J., Drewnick, F., Borrmann, S., Weimer, S., Demerjian, K., Salcedo, D., Cottrell, L., Griffin, R., Takami, A., Miyoshi, T., Hatakeyama, S., Shimono, A., Sun, J. Y., Zhang, Y. M., Dzepina, K., Kimmel, J. R., Sueper, D., Jayne, J. T., Herndon, S. C., Trimborn, A. M., Williams, L. R., Wood, E. C., Middlebrook, A. M., Kolb, C. E., Baltensperger, U., and Worsnop, D. R.: Evolution of organic aerosols in the atmosphere, Science., 326, 1525–1529, 2009.
Kleeman, M. J., Riddle, S. G., Robert, M. A., and Jakober, C. A.: Lubricating oil and fuel contributions to particulate matter emissions from light-duty gasoline and heavy-duty diesel vehicles, Environ. Sci. Technol., 42, 235–242, 2008.
Kroll, J. H. and Seinfeld, J. H. Chemistry of secondary organic aerosol: Formation and evolution of low-volatility organics in the atmosphere, Atmos. Environ., 42, 3593–3624, 2008.
Lim, Y. B. and Ziemann, P. J.: Effects of molecular structure on aerosol yields from OH radical-initiated reactions of linear, branched, and cyclic alkanes in the presence of NOx, Environ. Sci. Technol., 43, 2328–2334, 2009.
Liu, Z. and Phillips, J. B.: Comprehensive two-dimensional gas chromatography using an on-column thermal modulator interface, J. Chromatogr. Sci., 29, 227–231, 1991.
May, A. A., Presto, A. A., Hennigan, C. J., Nguyen, N. T., Gordon, T. D., and Robinson, A. L.: Gas-particle partitioning of primary organic aerosol emissions: (2) Diesel vehicles, Environ. Sci. Technol., 47, 8288–8296, 2013.
McNutt, M. K., Chu, S., Lubchenco, J., Hunter, T., Dreyfus, G., Murawski, S. A., and Kennedy, D. M.: Applications of science and engineering to quantify and control the Deepwater Horizon oil spill, P. Natl. Acad. Sci. USA, 109, 20222–20228, 2012.
Phillips, J. B. and Xu, J.: Comprehensive multi-dimensional gas chromatography, J. Chromatogr. A, 703, 327–334, 1995.
Reddy, C. M., Arey, J. S., Seewald, J. S., Sylva, S. P., Lemkau, K. L., Nelson, R. K., Carmichael, C. A., McIntyre, C. P., Fenwick, J., and Ventura, G. T.: Composition and fate of gas and oil released to the water column during the Deepwater Horizon oil spill, P. Natl. Acad. Sci. USA, 109, 20229–20234, 2012.
Reichenbach, S. E., Kottapalli, V., Ni, M., and Visvanathan, A.: Computer language for identifying chemicals with comprehensive two-dimensional gas chromatography and mass spectrometry, J. Chromatogr. A, 1071, 263–269, 2005.
Riazi, M. R.: Characterization and properties of petroleum fractions, 1st ed., American Society for Testing Materials, West Conshohocken, Pennsylvania, USA, MNL50, 2005.
Robinson, A. L., Donahue, N. M., Shrivastava, M. K., Weitkamp, E. A., Sage, A. M., Grieshop, A. P., Lane, T. E., Pierce, J. R., and Pandis, S. N.: Rethinking organic aerosols: semivolatile emissions and photochemical aging, Science, 315, 1259–1262, 2007.
Rogge, W. F., Hildemann, L. M., Mazurek, M. A., Cass, G. R., and Simoneit, B. R. T.: Sources of fine organic aerosol. 2. Noncatalyst and catalyst-equipped automobiles and heavy-duty diesel trucks, Environ. Sci. Technol., 27, 636–651, 1993.
Sakurai, H., Tobias, H. J., Park, K., Zarling, D., Docherty, K. S., Kittelson, D. B., McMurry, P. H., and Ziemann, P. J.: On-line measurements of diesel nanoparticle composition and volatility, Atmos. Environ., 37, 1199–1210, 2003.
Schauer, J. J., Kleeman, M. J., Cass, G. R., and Simoneit, B. R. T.: Measurement of Emissions from Air Pollution Sources. 2. C1 through C30 Organic Compounds from Medium Duty Diesel Trucks, Environ. Sci. Technol., 33, 1578–1587, 1999.
Schauer, J. J., Kleeman, M. J., Cass, G. R., and Simoneit, B. R.: Measurement of emissions from air pollution sources. 5. C1–C32 organic compounds from gasoline-powered motor vehicles, Environ. Sci. Technol., 36, 1169–1180, 2002.
Sonntag, D. B., Bailey, C. R., Fulper, C. R., and Baldauf, R. W.: Contribution of lubricating oil to particulate matter emissions from light-duty gasoline vehicles in Kansas City, Environ. Sci. Technol., 46, 4191–4199, 2012.
Wang, F. C.-Y., Robbins, W. K., and Greaney, M. A.: Speciation of nitrogen-containing compounds in diesel fuel by comprehensive two-dimensional gas chromatography, J. Sep. Sci., 27, 468–472, 2004.
Weitkamp, E. A., Sage, A. M., Pierce, J. R., Donahue, N. M., and Robinson, A. L.: Organic aerosol formation from photochemical oxidation of diesel exhaust in a smog chamber, Environ. Sci. Technol., 41, 6969–6975, 2007.
Welthagen, W., Mitschke, S., Muhlberger, F., and Zimmermann, R.: One-dimensional and comprehensive two-dimensional gas chromatography coupled to soft photo ionization time-of-flight mass spectrometry: a two- and three-dimensional separation approach, J. Chromatogr. A, 1150, 54–61, 2007.
Worton, D. R., Isaacman, G., Gentner, D. R., Dallmann, T. R., Chan, A. W., Ruehl, C., Kirchstetter, T. W., Wilson, K. R., Harley, R. A., and Goldstein, A. H.: Lubricating oil dominates primary organic aerosol emissions from motor vehicles, Environ. Sci. Technol., 48, 3698–3706, 2014.
Worton, D. R., Zhang, H., Isaacman-VanWertz, G., Chan, A. W., Wilson, K. R., and Goldstein, A. H.: Comprehensive chemical characterization of hydrocarbons in NIST standard reference material 2779 Gulf of Mexico crude oil, Environ. Sci. Technol., 49, 13130–13138, 2015.
Zielinska, B., Sagebiel, J., McDonald, J. D., Whitney, K., and Lawson, D. R.: Emission Rates and Comparative Chemical Composition from Selected In-Use Diesel and Gasoline-Fueled Vehicles, J. Air Waste Manage., 54, 1138–1150, 2004.