the Creative Commons Attribution 4.0 License.
the Creative Commons Attribution 4.0 License.
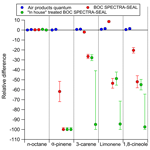
The importance of cylinder passivation for preparation and long-term stability of multicomponent monoterpene primary reference materials
Nicholas D. C. Allen
David R. Worton
Paul J. Brewer
Celine Pascale
Bernhard Niederhauser
Monoterpenes play an important role in atmospheric chemistry due to their large anthropogenic and biogenic emission sources and high chemical reactivity. As a consequence, measurements are required to assess how changes in emissions of monoterpenes impact air quality. Accurate and comparable measurements of monoterpenes in indoor and outdoor environments require gaseous primary reference materials (PRMs) that are traceable to the international system of units (SI). PRMs of monoterpenes are challenging to produce due to the high chemical reactivity and low vapour pressures of monoterpenes and also their propensity to convert into other compounds, including other terpenes. In this paper, the long-term stability of gravimetrically prepared static monoterpene PRMs produced in differently passivated cylinders, including sampling canisters, was assessed. We demonstrate that static PRMs of multiple monoterpenes can be prepared and used as a suitable long-term standard. For the first time the effect of cylinder pressure and decanting from one cylinder to another on the chemical composition and amount fraction of monoterpenes was also studied. Gravimetrically prepared PRMs of limonene in high pressure cylinders were compared to a novel portable dynamic reference gas generator based on dilution of pure limonene vapour emitted from a permeation tube.
- Article
(1242 KB) - Full-text XML
-
Supplement
(786 KB) - BibTeX
- EndNote
Terpenes are a large and diverse family of naturally occurring organic compounds that are a major biosynthetic building block (de Meijere et al., 1998; Nicklaus et al., 2013). Vegetation including forests and agricultural crops (Curtis et al., 2014; Ormeño et al., 2010) emit substantial quantities of isoprene (a hemiterpene (C5H8)), monoterpenes (C10H16) and sesquiterpenes (C15H24) (Barkley et al., 2008; Jokinen et al., 2015; Smolander et al., 2014; Squire et al., 2014; Tao and Jain, 2005).
Terpenes play an important role in atmospheric chemistry due to their high reactivity influencing the HOx and NOx budgets (Carslaw et al., 2017; Forester and Wells, 2011; Ng et al., 2007; Presto et al., 2005; Riu-Aumatell et al., 2004). The photochemical reactions of terpenes can lead to the production of tropospheric ozone, which is highly toxic to humans (Wolkoff et al., 2000), and the formation of secondary organic aerosol with implications for climate (Coleman et al., 2008; Lee et al., 2006; Ng et al., 2007; Vibenholt et al., 2009).
Terpenes are also known to be emitted from building materials and household products (Allen et al., 2016), in which they are primarily used as fragrances and flavourings (Lamorena and Lee, 2008; Steinemann et al., 2011; Wang et al., 2017; Wolkoff et al., 1998), impacting indoor air quality (Nazaroff and Goldstein, 2015; Singer et al., 2006). In particular, the exposure of the public to terpenes in indoor air quality is poorly understood due to a lack of available data, despite the toxicity of their photochemical products (Jones, 1999; Wolkoff and Nielsen, 2001; Wang et al., 2007; Wang et al., 2017).
A variety of techniques have been used for the sampling and analysis of complex mixtures of terpenes including active and passive sorbent tube loading and desorption (Sunesson et al., 1999), canister sampling (Batterman et al., 1998; Pollmann et al., 2005) followed by analysis using gas chromatography mass spectrometry (Birmili et al., 2003; Koch et al., 2000), proton transfer reaction mass spectrometry (Holzinger et al., 2005) or other spectroscopic techniques (Qiu et al., 2017). However, the accurate measurement of terpene amount fractions in indoor and outdoor air is highly dependent upon the availability of appropriate SI traceable gaseous PRMs (Rhoderick, 2010) and analytical methods (Helmig et al., 2013).
The World Meteorology Organisation (WMO) Global Atmosphere Watch (GAW) programme is a framework to provide reliable scientific data and information on the long-term trends in the chemical composition of the atmosphere. In WMO-GAW report no. 171 Global Long-Term Measurements of Volatile Organic Compounds (VOCs), new data quality objectives were created for priority VOC compounds including monoterpenes. These data quality objectives stipulated 20 % accuracy and 15 % precision for monoterpene measurements reported by GAW stations. Further recommendations by GAW's scientific advisory group for reactive gases have been made to lower these data quality objectives to 5 % and renamed as uncertainty and repeatability (Hoerger et al., 2015). In order to meet the 5 % uncertainty target, and prevent the reference material from dominating the uncertainty, stable PRMs of monoterpenes with uncertainties of better than 1.25 % (less than a quarter of the uncertainty) are required. There is also a requirement for performing reliable sampling or dynamic calibration methods for the in situ calibration of instruments during field campaigns or at long-term atmospheric monitoring stations and for independent verification of the gaseous PRMs.
PRMs containing monoterpenes are challenging because monoterpenes are highly reactive compounds and can isomerise, tautomerise or react to form a wide range of other compounds including other terpenes (Allahverdiev et al., 1998; Findik and Gunduz, 1997; Foletto et al., 2002). This has led to observations that the amount fraction of some monoterpenes increase overtime, including the observation of compounds that were not present when the mixture was first prepared, while the amount fraction of others declines (Rhoderick and Lin, 2013). Moreover, cylinder passivation (the coating applied to the internal surface of a cylinder to reduce adsorptive losses) has a big impact on the stability of monoterpene gas mixtures. Rhoderick and Lin (2013) demonstrated that specific passivation types, such as “Experis” (Quantum) manufactured by Air Products, looked the most promising for monoterpenes.
In this paper, multicomponent monoterpene static gaseous PRMs containing α-pinene, 3-carene, R-limonene and 1,8-cineole, as well as a mixture containing β-pinene were prepared gravimetrically at high pressure in cylinders with different internal surface passivations. The effects of adsorption on the cylinder walls and the cylinder pressure were assessed through a series of decanting experiments for these different cylinder passivations. The monoterpene PRM in the most suitable cylinder passivation treatment was analysed repeatedly over a 2-year period to assess the long-term stability building on the previous shorter-term stability studies of Rhoderick (2010) and Rhoderick and Lin (2013). The PRM containing limonene was compared to a new dynamic system based on permeation known as Reactive Gas Standard 2 (ReGaS2) developed by the Federal Institute of Metrology (METAS; Pascale et al., 2017), that is based on the dynamic dilution of limonene from a permeation tube to evaluate any systematic biases between the two different approaches. A portion of a monoterpene PRM was decanted into SilcoNert 2000® (Silcotek) treated sampling canisters to study their stability and suitability for short-term storage after field sampling.
2.1 Gravimetric preparation of PRMs
PRMs containing the four monoterpenes, α-pinene (both the minus and plus optical isomers), 3-carene, R-limonene and 1,8-cineole, as well as one containing n-octane (used as an internal reference standard), were prepared independently in a balance of high purity dry nitrogen (BIP+, Air Products) in accordance with ISO 6142 (ISO, 2015). Each monoterpene compound was prepared gravimetrically as a binary mixture (mixtures A–E) at an amount fraction of nominally 5–10 µmol mol−1 by liquid injection of each monoterpene, via a transfer vessel, into individual 10 L evacuated cylinders ( mbar). A balance of high purity nitrogen (BIP+, Air Products) was added by direct filling through an additional purifier (Microtorr, SP600F, SAES Getters) to remove trace impurities to below <1 nmol mol−1, such as hydrocarbons and water. Two β-pinene mixtures were also produced in a similar way (mixtures F and G). The compound and the amount fraction of the parent PRMs were: limonene 4.968±0.044 µmol mol−1 (mixture A), -pinene 9.942±0.029 µmol mol−1 (mixture B), 1,8-cineole 5.007±0.028 µmol mol−1 (mixture C), 3-carene 4.954±0.036 µmol mol−1 (mixture D), n-octane 9.995±0.038 µmol mol−1 (mixture E), -pinene 9.829±0.090 µmol mol−1 (mixture F) and 10.492±0.175 µmol mol−1 (mixture G) with all uncertainties in the gravimetric preparation expanded (k=2).
All “pure” liquid compounds were purchased from commercial suppliers (Fluka and Sigma Aldrich) and were purity analysed following the guidelines stipulated in ISO 19229:2015 by gas chromatography with a flame ionisation detector (GC-FID) prior to use. Impurities were identified and quantified by percentage area. The purity of all the monoterpenes was between 93.5 % and 99.5 % (Table S1 in the Supplement).
A PRM of nominally 100 nmol mol−1 (mixture AA, see Table 1) containing the four monoterpenes and n-octane was prepared by direct transfer of a portion (10–25 g) of each gravimetrically prepared parent mixture (A–E) and topped up with a balance of filtered high purity dry nitrogen (BIP+, Air Products) that was again added by direct filling through the purifier. A final dilution stage was carried out to prepare a PRM at nominally 2 nmol mol−1 (mixture BB, Table 1). A second nominal 2 nmol mol−1 mixture (mixture CC) was prepared in the same way to mixture BB for the long-term stability comparison. All of the PRMs were prepared in 10 L Experis passivated cylinders from Air Products, Belgium.
2.2 Analytical set-up
All of the measurements were performed using a GC-FID (Varian CP-3800). The system uses a sample pre-concentration trap containing glass beads cooled by liquid nitrogen and held at −100 ∘C during sampling to collect and focus the analytes prior to injection and separation on a GC column (Varian CP-Sil 13; 75 m × 0.53 mm, phase thickness = 2.0µm). All mixtures were connected to the GC using SilcoNert 2000® passivated stainless steel tubing. The lines were thoroughly purged and flow rates were allowed to stabilise for at least 10 min before commencing analysis.
The PRMs were connected to the GC using a minimal dead volume connector and the flow rate was set to 50 mL min−1 using a custom flow restrictor. For the dynamic ReGaS2 system a flow of 50 mL min−1 could not be achieved. Consequently, the volume flowed across the trap was recorded by a mass flow meter, calibrated with nitrogen, and subsequently corrected to match the sample volume of the high pressure gas standards. Mixtures were compared by running a series of up to six replicate analyses in blocks with the unknown mixture being analysed between two blocks of the PRM mixture to correct for any instrumental drift during analysis. The observed relative standard deviations in the peak areas of all compounds were between 0.3 % and 1.5 %.
2.3 Decanting experiments
A schematic illustrating the decanting procedure is shown in Fig. 1. The decanting experiments were performed in 10 L aluminium Luxfer cylinders that had been treated with different types of cylinder passivation, these included Experis, sometimes referred to as Quantum (Air Products), SPECTRA-SEAL (BOC) and “in-house” treated BOC SPECTRA-SEAL. It has been observed that this propriety “in-house” passivation provides improved stability for a wide range of compounds at low amount fractions. All cylinders had a 10 L internal volume. Initially, a new PRM, identified as cylinder 1 in Fig. 1 was prepared gravimetrically (as described in Sect. 2.2) at an amount fraction of nominally 2 nmol mol−1 and a pressure of 120 bar (cylinder 1) from a dilution of a 100 nmol mol−1 PRM (mixture AA).
Once a new PRM (cylinder 1) had been prepared at 120 bar (day 1), the mixture was analysed by GC-FID and compared against the reference PRM, mixture BB (day 2). The following day (day 3), approximately 50 bar of cylinder 1 was decanted by direct fill (a short well-purged transfer line) to cylinder 2 leaving 70 bar in cylinder 1. Both cylinder 1 and 2 were then analysed by GC-FID and compared against reference PRM, mixture BB. Finally (day 4), approximately 20 bar of cylinder 2 was decanted to cylinder 3 leaving 30 bar in cylinder 2 and both cylinder 2 and 3 were then analysed by GC-FID and compared against reference PRM, mixture BB (differences in the gravimetric values between the PRM and the reference standard were normalised). All of the cylinders were evacuated and the decant procedure was repeated for a second time.
All of the analyses were performed using GC-FID as described in Sect. 2.2. The amount fraction of each compound in the decanted cylinder was determined through a comparison with a nominal 2 nmol mol−1 reference PRM (mixture BB). If there were no losses, then the amount fraction of the decanted cylinders would be the same as those of the PRM cylinder 1. Decant losses were determined for each compound by calculating the relative difference between the amount fraction (AFdecant) of each compound in the decanted mixture and the expected amount fraction of that compound (AFexpected), which was defined as its gravimetric value before any decanting:
The amount fraction of each compound after decanting (AFdecant) was calculated from
where, Areaavgdecant was the average peak area for a set of GC runs (typically five) of the decanted mixture, AreaavgBB was the average peak area for a set of GC runs of in-house reference PRM, mixture BB, and GravBB is the gravimetrically assigned value of the compound in mixture BB.
2.4 Short and long-term stability study of monoterpene PRMs
To determine the short and long-term stability of the four component monoterpene reference PRM, mixture BB was regularly analysed over a 3-month (75 day) period. GC peak area responses of each terpene were ratioed to n-octane, which is known to be stable in this passivation type for more than 2 years (Grenfell et al., 2010) and was present in the mixtures as an internal standard. The long-term stability of mixture BB (prepared on 2 June 2015) was determined by preparing a fresh nominal 2 nmol mol−1 mixture (mixture CC), prepared 2.5 years later (904 days) on the 22 November 2017, and comparing the peak areas and their response factors.
β-Pinene, which is known to decompose over time in the presence of other terpenes (Foletto et al., 2002), was prepared at 10 µmol mol−1 in 2015. An independently prepared β-pinene binary was prepared 2.5 years later and the areas and response factors were compared to determine stability.
2.5 Canister experiment
A large number of samples are collected in the field during measurement campaigns. It is imperative that these samples can be collected and stored in a way that preserves the contents until they are analysed. One commonly used option is the use of sampling canisters or vessels that have been evacuated prior to use. Previous work has shown that the use of stainless steel canisters for sampling terpenes in dry or humidified air can be problematic (Batterman et al., 1998). Here we decant a portion of our 2 nmol mol−1 in-house reference PRM (mixture BB) into a SilcoNert 2000® treated 2 L sampling canister to determine their suitability for short-term storage of monoterpenes. The content was analysed by GC-FID and compared against the same nominal 2 nmol mol−1 reference PRM (mixture BB) to determine if any losses were observed over a 3-month period (83 days).
2.6 ReGaS2 dynamic system
An alternative to PRM preparation in high pressure cylinders is dynamic preparation using permeation. The ReGaS2 is a mobile generator that can produce traceable reference gas mixtures of a number of species, including terpenes (Pascale et al., 2017).
The method is based on permeation and subsequent dynamic dilution: a permeation tube containing the pure terpene is stored in an oven used as permeation chamber. The pure substance permeates at a constant rate into the matrix gas and can be diluted to give the desired amount fraction. The mass loss over time of the permeation tube is precisely calibrated using a traceable magnetic suspension balance. All parts in contact with the reference gas are coated with SilcoNert2000®.
The ReGaS2 mobile gas generator was fitted with a limonene permeation tube and set to dynamically generate an output of nominally 4 nmol mol−1. The amount fraction of the limonene produced by the dynamic system was measured using the same analytical set-up as described in Sect. 2.2 and compared to our nominal 2 nmol mol−1 reference PRM (mixture BB).
2.7 Uncertainty calculations
The evaluation of measurement uncertainty was in accordance with the “Guide to the expression of uncertainty in measurement” (Joint Committee for Guides in Metrology, 2008).
In the Supplement there is a description of an uncertainty evaluation when comparing the response of an unknown mixture against a validated calibration standard, e.g. a PRM (Eqs. S1–S4).
3.1 Decanting experiments and selection of passivation treatment for long-term stability measurements
The adsorption of the monoterpenes to the internal surfaces of the cylinder and valve were investigated through a series of decanting experiments as detailed in Sect. 2.3. The results for the different passivation types at 120 bar are shown in Fig. 2. There is a tabulated summary of the results of the decanting experiments in Tables S2–S7.
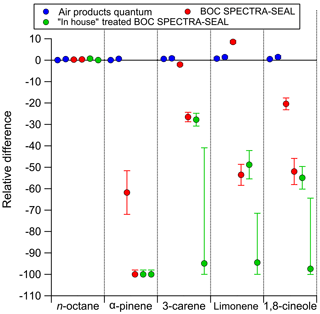
Figure 2The relative difference between the amount fraction of the decanted mixtures and the expected amount fraction based on gravimetric value of the mixture before any decanting. Each decantation was performed twice for each passivation type.
Decant losses of monoterpenes in the 10 L cylinders internally passivated with Air Products Experis treatment were minimal (Tables S2 and S3). No statistically significant differences were observed, therefore it can be confirmed, in agreement with Rhoderick and Lin (2013) that Experis cylinders are the most suitable for containing monoterpene PRMs. Figure 3 shows that the amount fraction does not appear to be influenced by the pressure within the cylinder, down to low pressure at 30 bar, as all agree within the measurement uncertainty and there is no overall directional trend. Below 30 bar we observe that the ratio is less than 1 for all components. While the results are within the measurement uncertainty, wall factors could have an influence on composition at low pressures (<30 bar) (Brewer et al., 2018). As reported in Brewer et al. (2018) compounds adsorbed to the walls at high pressure were observed to desorb back into the gas phase at lower pressures.
Figure 2 and Tables S3 and S4, show the initial decant, and repeat decant at 120 bar, in 10 L cylinders passivated internally with BOC SPECTRA-SEAL treatment. Aside from the n-octane a significant decrease in the amount fraction of all monoterpenes was observed (except for limonene in the first decant) relative to the reference PRM (BB). No further decants were performed for this cylinder type as the passivation was shown to be unsuitable for monoterpenes, with strong degradation observed by GC (Fig. 4) within less than 24 h after making the initial PRM. In an attempt to improve the stability of trace monoterpenes in SPECTRA-SEAL passivated cylinders, further in-house treatment was applied to a new set of cylinders. The results of this are shown in Fig. 2 and Tables S5 and S6, however no improvement was observed and all of the monoterpenes showed significant losses when the PRM was analysed by GC, less than 24 h after preparation.
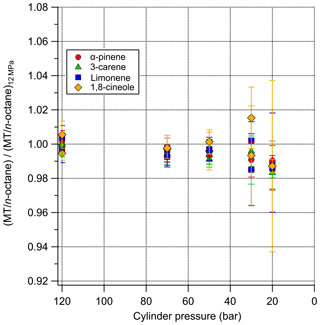
Figure 3The relationship between cylinder pressure and monoterpene amount fraction after normalisation to n-octane.
To investigate potential degradation components, a sample of a monoterpene mixture in an internally treated SPECTRA-SEAL cylinder was loaded onto a set of Chromasorb-106 and Tenax sorbent tubes (both packed in-house) and analysed on a Thermal-Desorption Gas Chromatograph Mass Spectrometer (TD-GC-MS). Similarly, a portion of the reference PRM (mixture BB) was also loaded onto Chromasorb-106 and Tenax sorbent tubes and analysed by TD-GC-MS. Five major peaks were consistently observed in the chromatograms of the desorbed tubes (Fig. 4). The additional peaks observed in the sample from the SPECTRA-SEAL cylinder were identified as the following monoterpenes: (a) α-terpinene, (b) τ-terpinene, (c) terpinolene, (d) cymene and (e) camphene, based on retention time and MS library matching to the NIST database. Mass spectrometry was used for compound identification and good forward match (FM) and reverse match (RM) values, predominantly >900 and all above 860 were obtained (see Tables S8 and S9 for details of the elution times, FM and RM values and Fig. S1 for mass spectra).
Interestingly, α-terpinene is produced industrially by acid-catalysed rearrangement of α-pinene and camphene by oxidation of α-pinene (Findik and Gunduz, 1997), which had disappeared from the SPECTRA-SEAL passivated cylinders after 24 h (Fig. 4). No other terpenes or peaks were observed in the sample of the reference PRM (mixture BB) except for the expected α-pinene, 3-carene, R-limonene and 1,8-cineole and n-octane. Kovats' retention indices were used to confirm the assignment of terpene compounds (Table S10 and Fig. S2).
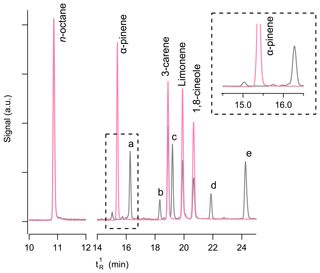
Figure 4Typical chromatograms for a stable (pink) and an unstable (grey) terpene mixture. The nominally 2 nmol mol−1 reference PRM, mixture BB (shown in pink) in an Air Products Experis cylinder was made from the same parent PRM as the PRM made in the internally passivated BOC SPECTRA-SEAL cylinder (shown in grey). The SPECTRA-SEAL cylinder was analysed less than 24 h after preparation and shows significant degradation of the terpene compounds. The zoomed in portion of the chromatogram focuses on the α-pinene peak (inset), showing that all of this compound has been lost. The additional peaks observed in the analysis of the SPECTRA-SEAL passivated cylinder, labelled as a–e, correspond to those named in the main text and to the observed MS shown in Fig. S1.
3.2 Short- and long-term stability study of monoterpene PRM
The short-term and long-term stability of mixture BB was determined through a series of experiments as detailed in Sect. 2.4. Over the first 3-month period that mixture BB was analysed the ratio of the monoterpene to n-octane remains constant within the measurement uncertainty. Regression analysis using a least squares fit shows that the gradients for all four monoterpenes are within the measurement uncertainty of zero showing no statistically significant change in amount fraction over the 75 day timeframe.
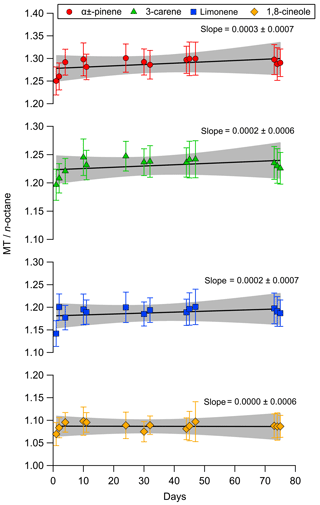
Figure 5The short-term stability of reference PRM (mixture BB) at nominally 2 nmol mol−1 compared as a ratio of the area of each monoterpene normalised relative to the n-octane internal standard. Error bars are included to account for the relative standard deviation of the mean (k=2). The solid lines show the results of a linear least squares fitting routine with the shaded area showing the confidence interval (95 %) of the fit.
Mixture BB was prepared on 2 June 2015 and mixture CC was more than 2 years later (904 days) on the 22 November 2017. A set of measurements were run to compare mixture BB and CC. This was repeated twice in the space of 2 days. Gravimetric values were normalised and the peak areas of the monoterpenes were then compared and the differences recorded (Table 2). It was found that, unsurprisingly, n-octane shows the best agreement and smallest difference, however, all the monoterpenes agreed well and differences were no greater than 2.5 % between mixtures BB and CC. The relative standard deviations of the peak areas was between 0.1 % and 1.5 % with the larger relative standard deviations correlating to the highest differences between the gas mixtures suggesting that the measurement is one of the largest sources of uncertainty in the experimental differences. The comparison infers that the monoterpene mixtures in Experis treated cylinders are stable for over 2.5 years.
Mixture F and G containing β-pinene were prepared 976 days apart (approximately 2 years and 8 months difference), and were compared. Agreement for β-pinene, once normalised to take into account gravimetric differences, was better than 0.5 % and the relative standard deviation in the peak areas were 0.7 %–1.1 %. No systematic bias was observed. This suggests that in Experis treated cylinders there is little or no decay of β-pinene at the µmol mol−1 level when prepared as a binary mixture. Stability has been demonstrated for greater than 2.5 years suggesting that it is the interaction of β-pinene with other monoterpenes in multicomponent gas standards that is the likely cause of their degradation.
Table 2Comparison showing the percentage difference between PRM mixtures prepared more than 2 years apart to assess the long-term stability of mixture BB and mixture F. Gravimetric values were normalised and the peak areas compared. There are two columns for the comparison of mixture BB and CC as the comparison was repeated on 2 consecutive days.
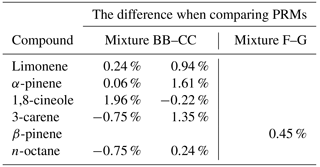
3.3 Short-term stability of monoterpenes in treated sampling canisters
Field campaign measurements require the short-term storage of VOC samples. Sampling canisters made from electropolished steel are frequently used despite losses being observed (Batterman et al., 1998). Another solution is to use SilcoNert 2000® treated canisters (silanisation treatment, Silcotek). However, the SPECTRA-SEAL cylinders that performed poorly in the decant experiments also use a silanisation surface treatment, therefore it was important to determine the suitability of SilcoNert 2000® treated canisters for short-term storage of monoterpenes. Following decant of mixture BB into the SilcoNert 2000® treated canister the contents were compared against mixture BB after 1, 8 and 83 days. The results of this are shown in Fig. 6.
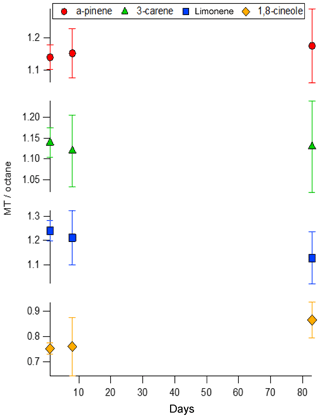
Figure 6The short-term stability of mixture BB decanted into a SilcoNert 2000® treated canister compared as a ratio of the area of each monoterpene normalised relative to the n-octane internal standard. Error bars are included to account for the relative standard deviation of the mean (k=2).
No statistically significant trends were observed for the stability, although higher than normal relative standard deviations in the GC peak areas were observed (≤4 % for all components except 1,8-cineole which was ≤8 %). This can be attributed to changes in the flow of gas from the canister samples during measurement due to the small volume and thus the decreasing pressure of the gas contained.
It appears that unlike the SPECTRA-SEAL passivated cylinders, the SilcoNert 2000® treated canisters would allow the storage of multi-component monoterpene standards for up to 3 months and still meet the data quality objective criteria recommended by GAW and its scientific advisory group (Hoerger et al., 2015). Nevertheless, this does not mean that a whole air sample containing terpenes or a broad array of terpenes together would behave in the same way due to the impact of humidity, therefore more work is required to determine this. However, it would suggest that decanting of PRMs for transport into the field in small SilcoNert 2000® treated canister should be possible.
3.4 Comparison of dynamic and static PRM
Two SI traceable preparation techniques for producing reference gas mixtures were compared. One was the preparation of static gravimetric PRMs, the other the generation of a dynamic reference standard from ReGaS2 using a permeation tube. From the weighing of the limonene permeation tube and from the data that was logged for the nitrogen flow and subsequent dilution it was calculated that the ReGaS2 mobile gas generator was outputting 4.41±0.32 nmol mol−1 of limonene with an expanded uncertainty of 7.3 % (k=2). Using the PRM static standards gravimetrically produced the output of the ReGaS2 dynamic system was estimated to be 3.57±0.11 nmol mol−1 of limonene with an expanded uncertainty of 2.9 % (k=2).
The static PRM that was used in this comparison (mixture BB) was also one of the mixtures used as part of the CCQM-K121 monoterpene key comparison at nominally 2.5 nmol mol−1. Results from CCQM-K121 demonstrated that all of the participants (Korea Research Institute of Standards and Science, KRISS; National Institute of Standards and Technology, NIST; and NPL) agree within the k=2 expanded uncertainties for all of the monoterpenes evaluated, including limonene.
One of the reasons for the systematic bias between the two approaches can be attributed to the temperature at which the permeator was operated, as the temperature was observed to have a strong influence on the reproducibility of the permeation rate. At lower temperatures, such as 30 ∘C (which was the temperature used for the comparison), the permeator does not reach a true steady state and it was observed that the variability on the permeation rate for the same temperature between two measurements was between 8 % and 10 %. A shift in the permeation rate of this magnitude coupled to uncertainties in temperature would be enough to compensate for the systematic bias observed between the two approaches.
The second reason is the 15 %–20 % decrease in the permeation rate. To investigate this further the permeation rate of limonene from the ReGaS2 dynamic system was measured over an 11-month period between March 2017 and February 2018. The decrease in the permeation rate was determined to be 35 % over this temporal period (Fig. S3) for the same temperature. The measurement of the permeation rate in the magnetic suspension balance lasted between 2 and 7 days with an associated uncertainty between 0.5 % and 1.5 % for one measurement at one temperature thus suggesting that the uncertainty assigned to ReGaS2 during the comparison was too low.
A decrease in the permeation rate of this magnitude coupled to the high uncertainties at such low temperatures would be enough to compensate for the systematic bias observed between the two approaches. Despite the systematic bias observed between the two methods at this trace level, the results of this first comparison are encouraging and show that state-of-the-art developments are being made with dynamic systems capable of delivering reliable outputs suitable for calibrating systems in the field.
In this paper we have investigated the short-term and long-term stability of monoterpenes in differently internally passivated cylinders. The choice of cylinder passivation is critically important in the preparation of monoterpene gas mixtures. We have demonstrated that Experis treated cylinders are the most appropriate for containing low amount fraction monoterpene PRMs and that the amount fraction is not influenced by pressure between 30 and 120 bar.
The need for suitable storage and transport of PRMs into the field has driven us to investigate the suitability of using SilcoNert 2000® treated canisters for monoterpenes. It was discovered that SilcoNert 2000® treated canisters could hold monoterpenes for up to 3 months with an uncertainty of 10 %, in line with GAW data quality objectives.
We compared the ReGaS2 dynamic mobile generator against high pressure static PRMs gravimetrically prepared at NPL. It was found that the output of limonene from dynamic ReGaS2 was 15 %–20 % lower than calculated. These differences correspond to less than 0.5 nmol mol−1 and it has been suggested that the bias may be attributed to the reproducibility of the limonene permeator at low temperature due to the permeation rate not reaching equilibrium. This first comparison of a dynamic terpene standard against a traditional static standard is the first step in providing the community with traceable reference materials suitable for in the field measurements to meet GAW data quality objectives.
PRM gravimetric data are provided in the paper. Decanting data are provided in the Supplement as percentages. GC-MS data were only used qualitatively; however, the spectra are also available in the supplement. Further information is available in the KeyVOCs EMRP final reports which can be found at: http://key-vocs.eu/impact (last access: 30 November 2018).
The supplement related to this article is available online at: https://doi.org/10.5194/amt-11-6429-2018-supplement.
NDCA and DRW wrote the paper. NDCA made the PRM gas mixtures, performed the labwork and designed the experiments. CP built the ReGaS2 dynamic system. DRW made the plots and led the decant work as part of the EMRP funded KeyVOC project. DRW, PJB and BN supervised. All authors discussed the results and commented on the manuscript.
The authors declare that they have no conflict of interest.
NPL and METAS were both funded as part of the European Metrology Research Programme (EMRP) “Metrology for VOC indicators in air pollution and climate change (KEYVOC)”. The EMRP is jointly funded by the EMRP participating countries within EURAMET and the European Union. Edited by: Eric C. Apel Reviewed by: two anonymous referees
Allahverdiev, A. I., Gunduz, G., and Murzin, D. Y.: Kinetics of alpha-pinene isomerization, Ind. Eng. Chem. Res., 37, 2373–2377, 1998.
Allen, N. D. C., Brewer, P. J., Brown, R. J. C., Lipscombe, R. P., and Woods, P. T.: International comparison of key volatile organic components in indoor air, Measurement, 82, 476–481, 2016.
Barkley, M. P., Palmer, P. I., Kuhn, U., Kesselmeier, J., Chance, K., Kurosu, T. P., Martin, R. V., Helmig, D., and Guenther, A.: Net ecosystem fluxes of isoprene over tropical South America inferred from Global Ozone Monitoring Experiment (GOME) observations of HCHO columns, J. Geophys. Res.-Atmos., 113, D20304, https://doi.org/10.1029/2008JD009863, 2008.
Batterman, S. A., Zhang, G. Z., and Baumann, M.: Analysis and stability of aldehydes and terpenes in electropolished canisters, Atmos. Environ., 32, 1647–1655, 1998.
Birmili, W., Berresheim, H., Plass-Dülmer, C., Elste, T., Gilge, S., Wiedensohler, A., and Uhrner, U.: The Hohenpeissenberg aerosol formation experiment (HAFEX): a long-term study including size-resolved aerosol, H2SO4, OH, and monoterpenes measurements, Atmos. Chem. Phys., 3, 361–376, https://doi.org/10.5194/acp-3-361-2003, 2003.
Brewer, P. J., Brown, R. J. C., Resner, K. V., Hill-Pearce, R. E., Worton, D. R., Allen, N. D. C., Blakley, K. C., Benucci, D., and Ellison, M. R.: Influence of Pressure on the Composition of Gaseous Reference Materials, Anal. Chem., 90, 3490–3495, 2018.
Carslaw, N., Fletcher, L., Heard, D., Ingham, T., and Walker, H.: Significant OH production under surface cleaning and air cleaning conditions: Impact on indoor air quality, Indoor Air, 27, 1091–1100, 2017.
Coleman, B. K., Lunden, M. M., Destaillats, H., and Nazaroff, W. W.: Secondary organic aerosol from ozone-initiated reactions with terpene-rich household products, Atmos. Environ., 42, 8234–8245, 2008.
Curtis, A. J., Helmig, D., Baroch, C., Daly, R., and Davis, S.: Biogenic volatile organic compound emissions from nine tree species used in an urban tree-planting program, Atmos. Environ., 95, 634–643, 2014.
de Meijere, A., Hadjiarapoglou, L., Noltemeyer, M., Gutke, H. J., Klein, I., and Spitzner, D.: Cyclopropyl building blocks for organic synthesis, 38 - Highly functionalized bicyclo 3.2.1 octane derivatives from readily available 2 '-alkoxytricyclo 3.2.1.0(2,7) octanes: Building blocks for terpenes, Part I, Eur. J. Org. Chem., 441–451, 1998.
Findik, S. and Gunduz, G.: Isomerization of alpha-pinene to camphene, J. Am. Oil Chem. Soc., 74, 1145–1151, 1997.
Foletto, E. L., Valentini, A., Probst, L. F. D., and Porto, L. M.: Gas-phase beta-pinene isomerization over acid-activated bentonite, Latin Am. Appl. Res., 32, 141–144, 2002.
Forester, C. D. and Wells, J. R.: Hydroxyl radical yields from reactions of terpene mixtures with ozone, Indoor Air, 21, 400–409, 2011.
Grenfell, R. J. P., Milton, M. J. T., Harling, A. M., Vargha, G. M., Brookes, C., Quincey, P. G., and Woods, P. T.: Standard mixtures of ambient volatile organic compounds in synthetic and whole air with stable reference values, J. Geophys. Res.-Atmos., 115, D14302, https://doi.org/10.1029/2009JD012933, 2010.
Helmig, D., Daly, R. W., Milford, J., and Guenther, A.: Seasonal trends of biogenic terpene emissions, Chemosphere, 93, 35–46, 2013.
Hoerger, C. C., Claude, A., Plass-Duelmer, C., Reimann, S., Eckart, E., Steinbrecher, R., Aalto, J., Arduini, J., Bonnaire, N., Cape, J. N., Colomb, A., Connolly, R., Diskova, J., Dumitrean, P., Ehlers, C., Gros, V., Hakola, H., Hill, M., Hopkins, J. R., Jäger, J., Junek, R., Kajos, M. K., Klemp, D., Leuchner, M., Lewis, A. C., Locoge, N., Maione, M., Martin, D., Michl, K., Nemitz, E., O'Doherty, S., Pérez Ballesta, P., Ruuskanen, T. M., Sauvage, S., Schmidbauer, N., Spain, T. G., Straube, E., Vana, M., Vollmer, M. K., Wegener, R., and Wenger, A.: ACTRIS non-methane hydrocarbon intercomparison experiment in Europe to support WMO GAW and EMEP observation networks, Atmos. Meas. Tech., 8, 2715–2736, https://doi.org/10.5194/amt-8-2715-2015, 2015.
Holzinger, R., Lee, A., Paw, K. T., and Goldstein, U. A. H.: Observations of oxidation products above a forest imply biogenic emissions of very reactive compounds, Atmos. Chem. Phys., 5, 67–75, https://doi.org/10.5194/acp-5-67-2005, 2005.
ISO: ISO 6142-1:2015 Gas analysis – Preparation of calibration gas mixtures – Part 1: Gravimetric method for Class I mixtures, International Organization for Standardization, 2015.
Joint Committee for Guides in Metrology: France Evaluation of measurement data – Guide to the expression of uncertainty in measurement (GUM 1995 with minor corrections), Working Group 1 of the Joint Committee for Guides in Metrology, 2008.
Jokinen, T., Berndt, T., Makkonen, R., Kerminen, V. M., Junninen, H., Paasonen, P., Stratmann, F., Herrmann, H., Guenther, A. B., Worsnop, D. R., Kulmala, M., Ehn, M., and Sipila, M.: Production of extremely low volatile organic compounds from biogenic emissions: Measured yields and atmospheric implications, P. Natl. Acad. Sci. USA, 112, 7123–7128, 2015.
Jones, A. P.: Indoor air quality and health, Atmos. Environ., 33, 4535–4564, 1999.
Koch, S., Winterhalter, R., Uherek, E., Kolloff, A., Neeb, P., and Moortgat, G. K.: Formation of new particles in the gas-phase ozonolysis of monoterpenes, Atmos. Environ., 34, 4031–4042, 2000.
Lamorena, R. B. and Lee, W.: Influence of ozone concentration and temperature on ultra-fine particle and gaseous volatile organic compound formations generated during the ozone-initiated reactions with emitted terpenes from a car air freshener, J. Hazard. Mater., 158, 471–477, 2008.
Lee, A., Goldstein, A. H., Kroll, J. H., Ng, N. L., Varutbangkul, V., Flagan, R. C., and Seinfeld, J. H.: Gas-phase products and secondary aerosol yields from the photooxidation of 16 different terpenes, J. Geophys. Res.-Atmos., 111, D17305, https://doi.org/10.1029/2006JD007050, 2006.
Nazaroff, W. W. and Goldstein, A. H.: Indoor chemistry: research opportunities and challenges, Indoor Air, 25, 357–361, 2015.
Ng, N. L., Chhabra, P. S., Chan, A. W. H., Surratt, J. D., Kroll, J. H., Kwan, A. J., McCabe, D. C., Wennberg, P. O., Sorooshian, A., Murphy, S. M., Dalleska, N. F., Flagan, R. C., and Seinfeld, J. H.: Effect of NOx level on secondary organic aerosol (SOA) formation from the photooxidation of terpenes, Atmos. Chem. Phys., 7, 5159–5174, https://doi.org/10.5194/acp-7-5159-2007, 2007.
Nicklaus, C. M., Minnaard, A. J., Feringa, B. L., and de Vries, J. G.: Synthesis of Renewable Fine-Chemical Building Blocks by Reductive Coupling between Furfural Derivatives and Terpenes, ChemSusChem, 6, 1631–1635, 2013.
Ormeño, E., Gentner, D. R., Fares, S., Karlik, J., Park, J. H., and Goldstein, A. H.: Sesquiterpenoid Emissions from Agricultural Crops: Correlations to Monoterpenoid Emissions and Leaf Terpene Content, Environ. Sci. Technol., 44, 3758–3764, 2010.
Pascale, C., Guillevic, M., Ackermann, A., Leuenberger, D., and Niederhauser, B. C.: Two generators to produce SI-traceable reference gas mixtures for reactive compounds at atmospheric levels, Meas. Sci. Technol., 124002, https://doi.org/10.1088/1361-6501/aa870c, 2017.
Pollmann, J., Ortega, J., and Helmig, D.: Analysis of atmospheric sesquiterpenes: Sampling losses and mitigation of ozone interferences, Environ. Sci. Technol., 39, 9620–9629, 2005.
Presto, A. A., Hartz, K. E. H., and Donahue, N. M.: Secondary organic aerosol production from terpene ozonolysis: 2. Effect of NOx concentration, Environ. Sci. Technol., 39, 7046–7054, 2005.
Qiu, C. L., Smuts, J., and Schug, K. A.: Analysis of terpenes and turpentines using gas chromatography with vacuum ultraviolet detection, J. Sep. Sci., 40, 869–877, 2017.
Rhoderick, G. C.: Stability assessment of gas mixtures containing terpenes at nominal 5 nmol/mol contained in treated aluminum gas cylinders, Anal. Bioanal. Chem., 398, 1417–1425, 2010.
Rhoderick, G. C. and Lin, J.: Stability Assessment of Gas Mixtures Containing Monoterpenes in Varying Cylinder Materials and Treatments, Anal. Chem., 85, 4675–4685, 2013.
Riu-Aumatell, M., Castellari, M., Lopez-Tamames, E., Galassi, S., and Buxaderas, S.: Characterisation of volatile compounds of fruit juices and nectars by HS/SPME and GUMS, Food Chem., 87, 627–637, 2004.
Singer, B. C., Destaillats, H., Hodgson, A. T., and Nazaroff, W. W.: Cleaning products and air fresheners: emissions and resulting concentrations of glycol ethers and terpenoids, Indoor Air, 16, 179–191, 2006.
Smolander, S., He, Q., Mogensen, D., Zhou, L., Bäck, J., Ruuskanen, T., Noe, S., Guenther, A., Aaltonen, H., Kulmala, M., and Boy, M.: Comparing three vegetation monoterpene emission models to measured gas concentrations with a model of meteorology, air chemistry and chemical transport, Biogeosciences, 11, 5425–5443, https://doi.org/10.5194/bg-11-5425-2014, 2014.
Squire, O. J., Archibald, A. T., Abraham, N. L., Beerling, D. J., Hewitt, C. N., Lathière, J., Pike, R. C., Telford, P. J., and Pyle, J. A.: Influence of future climate and cropland expansion on isoprene emissions and tropospheric ozone, Atmos. Chem. Phys., 14, 1011–1024, https://doi.org/10.5194/acp-14-1011-2014, 2014.
Steinemann, A. C., MacGregor, I. C., Gordon, S. M., Gallagher, L. G., Davis, A. L., Ribeiro, D. S., and Wallace, L. A.: Fragranced consumer products: Chemicals emitted, ingredients unlisted, Environ. Impact Asses., 31, 328–333, 2011.
Sunesson, A.-L., Sundgren, M., Levin, J.-O., Eriksson, K., and Carlson, R.: Evaluation of two adsorbents for diffusive sampling and thermal desorption-gas chromatographic analysis of monoterpenes in air, J. Environ. Monit., 1, 45–50, 1999.
Tao, Z. N. and Jain, A. K.: Modeling of global biogenic emissions for key indirect greenhouse gases and their response to atmospheric CO2 increases and changes in land cover and climate, J. Geophys. Res.-Atmos., 110, D21309, https://doi.org/10.1029/2005JD005874, 2005.
Vibenholt, A., Norgaard, A. W., Clausen, P. A., and Wolkoff, P.: Formation and stability of secondary ozonides from monoterpenes studied by mass spectrometry, Chemosphere, 76, 572–577, 2009.
Wang, C. M., Barratt, B., Carslaw, N., Doutsi, A., Dunmore, R. E., Ward, M. W., and Lewis, A. C.: Unexpectedly high concentrations of monoterpenes in a study of UK homes, Environ. Sci.-Proc. Imp., 19, 528–537, 2017.
Wang, S. B., Ang, H. M., and Tade, M. O.: Volatile organic compounds in indoor environment and photocatalytic oxidation: State of the art, Environ. Int., 33, 694–705, 2007.
Wolkoff, P., Schneider, T., Kildeso, J., Degerth, R., Jaroszewski, M., and Schunk, H.: Risk in cleaning: chemical and physical exposure, Sci. Total Environ., 215, 135–156, 1998.
Wolkoff, P. and Nielsen, G. D.: Organic compounds in indoor air – their relevance for perceived indoor air quality?, Atmos. Environ., 35, 4407–4417, 2001.
Wolkoff, P., Clausen, P. A., Wilkins, C. K., and Nielsen, G. D.: Formation of strong airway irritants in terpene/ozone mixtures, Indoor Air, 10, 82–91, 2000.
WMO: A WMO/GAW Expert Workshop on Global Long-Term Measurements of Volatile Organic Compounds (VOCs), WMO/TD – No. 1373, GAW Report No. 171, Geneva, Switzerland, 30 January–1 February 2006.