the Creative Commons Attribution 4.0 License.
the Creative Commons Attribution 4.0 License.
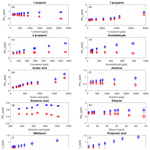
Negligible influence of livestock contaminants and sampling system on ammonia measurements with cavity ring-down spectroscopy
Jesper Nørlem Kamp
Albarune Chowdhury
Anders Peter S. Adamsen
Anders Feilberg
Emission of ammonia (NH3) is a ubiquitous problem due to the adverse effects of NH3 on the environment and human health. The agricultural sector accounts for nearly all NH3 emissions in Europe. Hence, technologies for the abatement of NH3 emissions from this sector have been in strong demand in recent years. In order to document emissions and evaluate abatement technologies, there is a strong need for reliable NH3 measurement methods. Photoacoustic spectroscopy (PAS) is often used to measure NH3 concentrations, but recent research shows interference from compounds typically present in livestock production and during agricultural activities. In this work, the performance of cavity ring-down spectroscopy (CRDS) from Picarro, as an alternative to PAS, has been tested with respect to method validation under laboratory and field conditions. Potential interferences of 10 volatile organic compounds (VOCs) on CRDS NH3 measurement were tested with simultaneous VOC analysis performed by proton-transfer-reaction mass spectrometry (PTR-MS). Both laboratory and field calibrations show excellent linearity over a large dynamic range of NH3 concentrations. The analyzer shows a small humidity effect of up to a few ppb in the extreme case of a nearly water-saturated air stream. Apart from the negligible humidity dependency, no interferences of the tested VOCs were observed. Overall, the CRDS system performs satisfactory and is well suited for measurements of NH3 emissions from livestock production.
- Article
(6406 KB) - Full-text XML
- BibTeX
- EndNote
Ammonia (NH3) is an important atmospheric pollutant with several adverse effects: deposition of NH3 can lead to eutrophication and acidification, which has negative effects on biodiversity (Sheppard et al., 2011). NH3 is a precursor of atmospheric aerosols thereby influencing the global radiation budget as well as having a negative influence on human health (Aneja et al., 2001; Baek et al., 2004). In addition, microbial oxidation of NH3 results in secondary production of nitrous oxide (N2O) and nitric oxide (NO) (Zhu et al., 2013). N2O is a very potent greenhouse gas (GHG) and NO is involved in atmospheric reactions producing tropospheric ozone (Aneja et al., 2001).
Agricultural activities, mainly manure application and management, account for around 94 % of NH3 emissions in Europe (Nielsen et al., 2017). Reliable measurements in the agricultural sector are highly important to give accurate estimates of NH3 emissions in order to reduce emissions, for example, by validation of technological improvements within the agricultural sector.
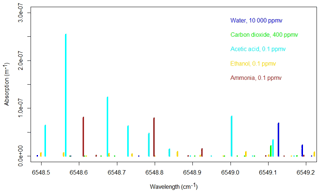
Figure 1Simulated absorption spectrum from the HITRAN database for 1 % H2O (blue), 400 ppm CO2 (green), 100 ppb acetic acid, 100 ppb ethanol, and 100 ppb NH3 at 45 ∘C and 187 mbar.
The ventilation air from animal production facilities contains a variety of chemical compounds, relatively high water content and high densities of particulate matter. This includes a great number of volatile organic compounds (VOCs) that are emitted in agricultural buildings from, e.g., silage, manure and the animals themselves (Feilberg et al., 2010; Hafner et al., 2013; Hansen et al., 2012; Ngwabie et al., 2008; Shaw et al., 2007; Yuan et al., 2017). Several NH3 analyzers are sensitive to water vapor and other gasses (Bobrutzki et al., 2010; Huszár et al., 2008; Ni and Heber, 2008; Rom and Zhang, 2010; Rosenstock et al., 2013). Such interferences can introduce errors depending on the instrument used. Furthermore, it is challenging to measure NH3 concentrations correctly due to its high water solubility and polarity, which causes adsorption on surfaces in the sampling system and within the instrument (Rom and Zhang, 2010; Shah et al., 2006; Vaittinen et al., 2014). This “sticky nature” of NH3 causes delays in the measurements, giving longer response times (Rom and Zhang, 2010; Shah et al., 2006; Vaittinen et al., 2014). It is an additional challenge to measure NH3 in livestock buildings, where dust and particles provide large surface areas for adsorption in particulate filters used to protect measuring instruments.
In many agricultural emission studies, photoacoustic spectroscopy (PAS) (Poissant et al., 2005; Rom and Zhang, 2010; Saha et al., 2010; Wu et al., 2012; Zhang et al., 2005; Zong et al., 2014) has been used, but findings by Rosenstock et al. (2013) and Liu et al. (2019) show high interference on NH3 measurements from a variety of organic compounds including carboxylic acids and alcohols. Hassouna et al. (2013) reported nonconstant bias in the results from PAS measurements on NH3 and N2O caused by organic compounds often present at agricultural sites, which makes the PAS measurements unreliable in an agricultural setting. Another issue with PAS measurements is the long response time of up to 25 min (Rom and Zhang, 2010), which lowers the time resolution of emission measurements under dynamic conditions. NH3 is underestimated by approximately 14 % and 2 % after 12.5 and 25 min, respectively (Rom and Zhang, 2010). Typical measurements in cattle barns take place at multiple points. For example, Rong et al. (2014) measured at 7 points in a dairy cow building and Ngwabie et al. (2009) measured at 11 points in a dairy cow barn. The cycle time for a typical setup in a barn would therefore be on the order of 3–5 h, which makes it impossible to monitor temporal variations with only 4–8 measurements per measurement point per day. Thus, the response time is a key parameter for equipment measuring at multiple points as done in livestock buildings.
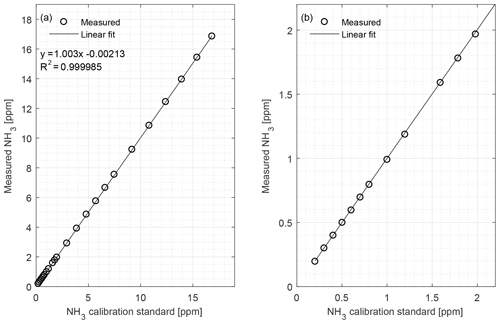
Figure 2(a) Calibration curve of the CRDS from 0.20 to 16.8 ppm NH3 conducted under laboratory conditions; (b) calibration curve limited to 0 to 2 ppm. Symbols represent measured values, error bars the standard deviation and the line is the fitted regression model.
In a few recent studies, cavity ring-down spectroscopy (CRDS) has been used to measure ammonia concentrations (Maasikmets et al., 2015; Sintermann et al., 2011) in agricultural settings. The CRDS provides measurements in real time with high sensitivity, high selectivity and a fast response time. The CRDS analyzer maintains high linearity, precision and accuracy over changing environmental conditions without the need for frequent calibration. The work of Martin et al. (2016) led to an improvement of water vapor interference calculations on Picarro's CRDS for NH3 measurements. The scaling factor error they discovered is approximately 2 % of the absolute water concentration.
Bobrutzki et al. (2010) conducted a field intercomparison of 11 atmospheric NH3 measurement techniques at concentrations up to 120 ppb including cavity ring-down spectroscopy (CRDS), and the results show a good overall agreement between the instruments on an hourly basis (R2>0.84).
Our aim is to demonstrate the performance of the CRDS analyzer for measurements of NH3 gas concentration under laboratory and field conditions. This includes identification and quantification of potential interferences by compounds present in livestock buildings by using proton-transfer-reaction mass spectrometry (PTR-MS) to document VOC concentrations in laboratory tests.
Due to the major concerns regarding measurements of NH3 with PAS (Hassouna et al., 2013; Liu et al., 2019; Zhao et al., 2012), it is pertinent that any influence of VOC on CRDS is thoroughly documented, which to our knowledge has not been done previously. The absorptions lines of, for example, acetic acid and methanol found in the HITRAN database (Gordon et al., 2017) are in the same range as the ammonia lines used for measurements in the CRDS; see Fig. 1. This highlights the importance of the current study as the absorption by VOCs in principle may cause similar interference, as reported by Rosenstock et al. (2013) and Liu et al. (2019) for PAS.
This study aims to validate CRDS for measurements in the agricultural industry, thus we test for interference with a number of compounds typically present in pig houses and cattle farms for which NH3 concentration and emission measurements are routinely carried out. Laboratory tests include determination of the response parameters linearity, response time, influence of particulate filters and chemical interference. Field tests include determination of the response parameters linearity, response time and particulate filter effect.
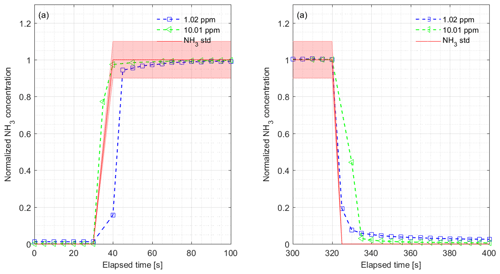
Figure 3(a) Rise time and (b) fall time for the CRDS measurements normalized to final concentrations during laboratory testing of the response to a step change to 1.02 (blue) and 10.01 ppm (green). The results show NH3 to 1.02 ppm with rise time s and fall time s and 10.01 ppm with rise time = 3.6 s and fall time = 4.8 s. The red lines and areas represent the NH3 standard gas concentration with 10 % accuracy, the blue markers show the normalized concentration from 1.02 ppm and the green markers show the normalized concentration from 10.01 ppm.
2.1 Chemicals, reagents and gasses
We used the following chemicals during the experiments: 70 mM acetic acid (VWR int. S.A.S., Fontenay-sous-Bois, France), 27 mM 1-propanol (Merck KGaA, Darmstadt, Germany), 1.3 mM 2-propanol (Sigma-Aldrich Chemie GmbH, Steinheim, Germany), 0.7 mM acetaldehyde (Sigma-Aldrich), 4 mM propionic acid (Alfa Aesar GmbH & Co KG, Karlsruhe, Germany), 0.8 mM acetone (Merck), 10 mM methanol (VWR), 2.2 mM 1-butanol (Merck), 69 mM ethanol (CCS Healthcare AB, Borlänge, Sweden) and 44 mM butanoic acid (Alfa Aesar). Deionized water dissolved the chemicals to the desired concentrations.
We used the following gasses during the experiments: 101 ppm NH3 (±10 %) in N2O calibration gas (AGA A/S, Copenhagen, Denmark), pure (99.99 %) CO2 (AGA), pure (99.99 %) CH4 (AGA), and as compressed zero air passed through a bed of silica gel and charcoal to remove water, ozone, hydrocarbons and other common contaminants. Mass flow controllers (MFCs) from the EL-FLOW (Bronkhorst High-Tech B.V., Ruurlo, Netherlands) series regulated all gas flows with an accuracy of ±5 %.
2.2 Instrumentation
The operational principle of CRDS relies on ring down time laser light. An air sample enters a cavity at low pressure (18.665 kPa, 140 Torr) and laser light is pulsed into the cavity, where almost all light is reflected by mirrors, which gives an effective path length of kilometers. A small fraction of the light penetrates the mirrors to reach the detector and the intensity of the light is proportional to the concentration of target gas, as the target gas will absorb light. The G2103 analyzer measures absorption from 6548.5 to 6549.2 cm−1 (Martin et al., 2016), and Fig. 1 shows the absorption of some selected compounds in this range obtained from the HITRAN 2016 database (Gordon et al., 2017). The computed absorption lines in Fig. 1 correspond to 1 % H2O, 400 ppm CO2, 100 ppb acetic acid, 100 ppb ethanol and 100 ppb ammonia at 45 ∘C and 140 Torr. Line broadening is not taken into account.
For the determination of interference, we used a Picarro NH3∕H2O analyzer model G2103 (Picarro Inc., Santa Clara, CA, USA) to measure the NH3 concentration continuously, this CRDS analyzer has not incorporated the upgraded water correction. A high-sensitivity PTR-MS (Ionicon Analytik, Innsbruck, Austria) measured concentrations of different VOCs for the interference tests. The drift tube setting was 600 V, 2.1–2.2 mbar and 60 ∘C, which yield an E/N of approximately 130 Td. Fragmentation of alcohols is normal in PTR-MS and we use the fragmentation of alcohols as described by Brown et al. (2010) to calculate the final concentration with all fragments taken into consideration.
One stream of clean air passed through the headspace air over an aqueous solution containing a single compound. Another stream diluted the outflow from the headspace. We changed the airflows to get different concentrations of the compound in the gas phase. The CRDS and PTR-MS received the diluted air streams.
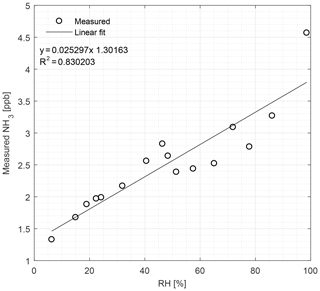
Figure 5The CRDS signals of NH3 (ppb) in zero air at different concentrations of water vapor, ranging from 6 % to 99 % relative humidity (RH) at 22 ∘C under laboratory conditions. Symbols represent measured values and the line is the fitted linear regression model.
2.3 Linearity, calibration and filter effect
We used a flow dilution system with zero air and NH3 calibration gas (101 ppm) to test the linearity of the CRDS measurements. NH3 gas concentrations for the calibration were in the range from 0.20 to 16.8 ppm in the laboratory and from 0.27 to 20.0 ppm under field conditions. We performed the calibrations in the laboratory without external filters. Introduction of all gasses in the field was through a multi-position rotary valve (MPV, Cheminert low-pressure valve, model C25, VICI AG International, Schenkon, Switzerland) for 6 min while the CRDS was in normal sampling mode. We performed a single point calibration in the field to test the system integrity and analyzer response time by introducing 7.8 ppm NH3 calibration gas directly into the sampling lines that were manually removed from their position. The response time for all experiments was found by fitting an exponential function to the step changes, which gave the e-folding time. We tested the PTFE filters in the laboratory for NH3 signals by connecting filters used for 2 weeks to a clean air supply under heating to maximum 75 ∘C. Monitoring of the NH3 signals continued until the concentration went below 5 ppb; see Table 1 for abbreviations and specifications of the used filters.
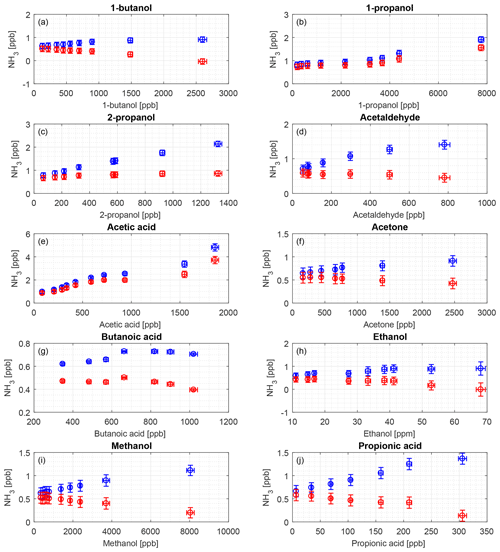
Figure 7Interference of different organic compounds on the CRDS NH3 measurement. Blue markers indicate the original data and red markers indicate water-corrected data from the regression shown in Fig. 5. The compounds are (a) 1-butanol, (b) 1-propanol, (c) 2-propanol, (d) acetaldehyde, (e) acetic acid, (f) acetone, (g) butanoic acid, (h) ethanol, (i) methanol, and (j) propionic acid.
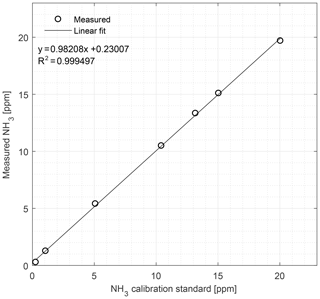
Figure 8Calibration curve of the CRDS from 0.27 to 20.04 ppm NH3 conducted under field conditions. Symbols represent measured values and the line is the fitted linear regression model.
We performed laboratory tests on the response time by switching between ambient air and 1.02 ppm NH3 with the MPV without external filters attached. We also tested response time to a step change in NH3 concentration with different external particulate filters attached. The concentrations were 0.203 and 10.01 ppm NH3 with filters of different pore sizes made of PTFE, glass fiber and quartz. Table 1 shows the specifications of the filters.
2.4 Field testing
We conducted field tests in a cattle barn with natural ventilation located in central Jutland outside Viborg, Denmark. The cattle barn is 9 m high, 60 m long and 36 m wide and naturally ventilated. We measured NH3 concentrations in the cattle building with the CRDS combined with a 10 port (P1-P10) MPV (C25-61800, VICI Valco Inst. Co. Inc., Texas, USA). Measurements were set up according to Rong et al. (2014) and Wu et al. (2012). We considered the division into three 20 m sections inside the cattle barn to be representative of the animal-occupied zone of the barn. We sampled NH3 concentrations from the three sections using PTFE tubes (inner diameter 6 mm, 20 m long) with 20 uniformly distributed sampling openings. The sampling points (SP) SP2, SP3 and SP4 were inside the building, with SP2 and SP4 on each of the end walls adjacent to the windows, i.e., sidewall openings, placed 2.5 m above the floor. SP3 was just below the ridge opening in the middle of the building, placed 9 m above the floor. SP1 and SP5 were outside background measurements from two single points placed 5 m from the building sidewalls at 2.5 m height. The sample tubes were between 5 and 50 m long with heating cables attached to avoid condensation inside the tubing. The length of the sampling lines was approximately 5, 15, 35, 45 and 50 m for SP1, SP2, SP3, SP4 and SP5, respectively. See Fig. A1 in the Appendix. Each sampling line had a secondary suction pump (flow rate of 6–7 L min−1) with a PTFE membrane to generate a constant flow through the lines. A PTFE filter (0.20 µm pore size) removed airborne particulate matter from the sample air before the sampling ports of the MPV. Replacement of filters was done at least fortnightly. Measurement lasted 6 min for each sampling port with automatic switching; i.e., a measurement cycle was 30 min.
We used pure deionized water to produce a range of different humidity levels. Figure 5 shows the effects of the humidity on the NH3 signal from relative humidity (RH) ranging from 6.3 % to 98.6 %. The response to the change in humidity is linear (R2=0.83) with NH3 measurements from 1.3 to 4.6 ppb over the given RH range.
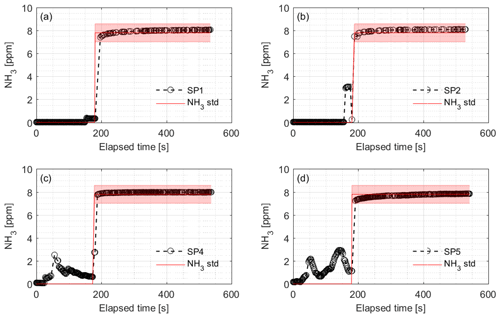
Figure 9Calibration of the NH3 sampling and measurement system and associated response times of the CRDS during field testing. Introduction of 7.8 ppm NH3 gas was at (a) SP1, (b) SP2, (c) SP4 and (d) SP5 while monitoring the NH3 concentration at the outlet port connected to the analyzer. SP denotes sampling point. The rise times were 7.3, 3.0, 8.4 and 5.9 s for SP1, SP2, SP4 and SP5, respectively. The red line and area represent the NH3 standard concentration with uncertainty.
3.1 Laboratory tests
The CRDS had a highly linear response (R2=0.99998) to NH3 concentrations over the dynamic range 0.20–16.8 ppm (Fig. 2). This range is chosen from the expected concentration in a livestock facility as seen in Figs. A2 and A3, which show the hourly mean concentration of NH3 in four rooms with finisher pigs and a dairy cattle barn, respectively. The maximum concentration can exceed 15 ppm in the pig houses and 3 ppm in the cattle barn. The NH3 standard calibration gas used for all calibrations had an accuracy of ±10 % stated by the manufacturer. The measured NH3 concentrations in Fig. 2 are averages of several hundred measurements and the standard deviations are indicators of stability. Figure 3a and b shows the result of a step change in concentration from clean air to 1.02 and 10.01 ppm, respectively, and back to clean air. The rise time to the final concentration level minus 1∕e was 8.1 and 3.6 s and the fall time to 1∕e of the final concentration level was 3.2 and 4.8 s for 1.02 and 10 ppm, respectively. Furthermore, Fig. 4 shows response times to step changes to two concentrations (0.203 and 10.01 ppm) with different types of external particulate filters. The response times varied for the different filter types, with an average rise and fall time of 8.1 and 6.3 s (for 0.203 ppm) and 3.1 and 3.7 s (for 10.01 ppm), respectively (Fig. 4). Across all filter types, the response time was fastest for changes to the highest concentration except the fall time for three filters; see details in Table A2.
Measurements on zero air over an hour gave a standard deviation on the NH3 concentration of 0.115 ppb. This gives a limit of detection (LOD) of 0.35 ppb for three standard deviations and a limit of quantification (LOQ) of 1.15 ppb for 10 standard deviations; see Table 2.
Figure 6 shows the CRDS signals from CO2 and CH4 with random fluctuations in the low ppb level being observed. There are no apparent interferences from these two compounds. All measured NH3 concentrations for both compounds are below the LOQ.
Figure 7 shows the interferences of 10 different VOCs with VOC concentration as a function of NH3 concentration corrected for water contribution and the uncorrected concentration as well. This correction was due to a clear pattern for increased water vapor with VOC concentration as water is introduced with the VOCs, and NH3 concentration increased with increased water vapor for all compounds. The observed interferences were in the range from 0.5 to 5 ppb NH3 equivalents at VOC concentrations from 6 to 8000 ppb. These VOC concentrations range from levels comparable to field conditions up to levels 1–2 orders of magnitude higher than maximum field conditions.
3.2 Field tests
In the field, the CRDS also has a highly linear response (R2=0.9995) in the concentration range 0.27–20.04 ppm; see Fig. 8. Figure 9 shows the results of a single point field calibration of the system integrity and response time to a sudden change to 7.8 ppm with response times varying from 6 to 25 s. The calibration gas used in the measurements shown in Figs. 8 and 9 had an uncertainty of ±10 %.
Figure 10 shows the ammonia concentrations released from external particulate filters that have been exposed to ammonia in a livestock house for 2 weeks. Vacuum pumps applied a gas flow rate of minimum 6 L min−1 through the filter over the 2-week period, thus a minimum volume of 120 m3 of air went through each filter. The preexposed filters were flushed with zero air and ammonia release was monitored by CRDS. The concentration maximum varied between 25 and 38 ppb. The peak values are comparable to typical ambient laboratory concentrations ranging from 14 to 37 ppb; see Table A1.
The CRDS analyzer had a linear response during both laboratory tests and field validation (Figs. 2 and 8) in the range from approximately 0.2 to 20 ppm; NH3 concentrations in livestock buildings are normally within this range (Heber et al., 2006; Koerkamp et al., 1998) as seen in Figs. A1 and A2. This is in agreement with the manufacturer specifications that guarantee the range from 0 to 500 ppb and an operational and optional expanded range up to 10 and 50 ppm, respectively (Picarro, 2017). The field calibrations show excellent agreement with the standard gas concentrations. The standard gas had an uncertainty of ±10 %, according to the data sheet from the manufacturer. This may well explain the small offset (< 4 %) from the obtained concentrations seen in Fig. 9, but the system causes minor variations, as the bias is considered constant. The LOD (0.35 ppb) found in the present study is comparable to the manufacturer's specifications for 1 s integration time, as seen in Table 2. The performance of a single point field calibration showed very good agreement with the expected concentrations, as seen in Fig. 9. The potential bias from the zero air might also influence the other laboratory experiments, which can explain some of the variations we see in, for example, filter response (Fig. 4) or water dependency (Fig. 5).
It is a requirement to have fast responding analyzers to understand the dynamic behavior and diurnal variations in NH3 concentrations in animal buildings. Ni and Heber (2008) suggest a response time of less than 2 min to capture temporal NH3 concentration variations. The CRDS shows sufficiently low response times under laboratory (Fig. 3 and Table A2) and field conditions (Fig. 9). These times are also comparable to < 30 s for responses to 3 ppm, as reported by the manufacturer (Picarro, 2017). Furthermore, there are no clear changes in response time without the use of an external particulate matter filter. The tested filters vary by < 10 s in response time with a tendency towards shorter response times at higher concentrations. The manufacturer reports rise- and fall times of approximately 16 s, which is at a minimum twice as much as the present results, Table A2 and Fig. 2. The response to a change from 0 to 1.02 ppm gave response times of 8.1 and 3.2 s for the rise and fall time, respectively. A change from 0 to 10.02 gave response times of 3.6 and 4.8 s for the rise and fall time, respectively (Fig. 3). The rise times with external particulate filters connected were 7.3, 3.0, 8.4 and 5.9 s, for SP1, SP2, SP4 and SP5, respectively. Response times are, in general, faster for higher concentration differences (see Fig. 4), which is ascribed to faster surface saturation. The observed concentrations of NH3 released from particulate filters exposed to air from a livestock house for 2 weeks (Fig. 10) suggest that adsorption of NH3 to the filter material, surfaces and walls is negligible. The levels released over 1 min (< 50 ppb) should be compared to a filter exposure of ammonia of > 100 ppb (ranging in to low ppm levels) over 2 weeks. These results indicate that the use of external filters gives satisfying response times and no problems with adsorption of NH3 on the filter material.
CO2 and CH4 are present in the atmosphere in relatively high concentrations compared to other trace gasses, and animals produce CO2 and CH4, thus elevated concentrations are normal in animal houses. Over a large concentration range, we observed little scatter and no interference of CO2 and CH4 on NH3 measurements, as seen in Fig. 6. The mean concentrations of both compounds are below the LOQ.
For the interference of single VOCs, it was expected that the different dilutions prepared from clean dry air mixed with humid headspace air over a VOC solution gave a correlation between water vapor and VOC concentration. This was also the case for all 10 volatile compounds. Martin et al. (2016) observe an interference from water vapor on NH3 measurements due to spectral line broadening, which the manufacturer corrects for in all models produced after the publication of that work. Our Picarro analyzer from December 2014 does not make this extra correction, and we thus expected a small water dependency for NH3, which is seen in Fig. 5. Figure 5 shows the humidity effect on the CRDS signal generated from pure deionized water and reveals a small dependency for water vapor, which the improvements suggested by Martin et al. (2016) potentially remove. Nonetheless, our results show up to 4.5 ppb NH3 for a nearly water-saturated air stream with an absolute H2O concentration of approximately 1.1 %. Thus, in the extreme case of low NH3 concentrations of 100 pbb and very humid air, a water vapor interference of up to 5 % of the NH3 signal may be present, but under normal conditions this is negligible.
The 10 tested compounds are normally present in sub-ppm levels in agricultural environments (Copeland et al., 2012; Yuan et al., 2017). As seen in Fig. 1, acetic acid and methanol have absorption lines in the wavelength area used for the Picarro CRDS measurements. A concentration range that covers a large dynamic area and exceeds the normal maximum concentration in livestock buildings was used to obtain the potential maximum interference, and we only observed very small water-induced interferences. Figure 7 shows the contribution from the single VOCs that were either corrected for water or uncorrected, and, as can be seen, the interferences are insignificant in general. Overall, the difference between high and low concentration for a single VOC was approximately 1–2 ppb NH3 (Fig. 7), except for acetic acid with a difference of nearly 4 ppb. It should be noted that the water to VOC relation differs for the different compounds. With water correction applied, only 1-propanol and acetic acid have increasing tendencies, where NH3 concentration increases about 0.8 ppb for an increase of 7.5 ppm of 1-propanol and 2.9 ppb for an increase of nearly 1.8 ppm of acetic acid. Acetic acid, 2-propanol and propionic acids were the only compounds with absolute humidity above 1 % as we used higher flow rates over the headspace to obtain the targeted concentrations. The very moist sample of acetic acid had a corrected maximum of 3.7 ppb NH3, which is very low compared to, for example, concentrations in animal buildings, which typically range from < 1 to 20 ppm but can, in extreme cases, reach up to 50 ppm (Heber et al., 2006; Koerkamp et al., 1998). Thus, errors of a few ppb, introduced by humidity effects, would have a small impact on the results. For the given setup, the interferences from water vapor were in the same order of magnitude as the LOQ of 1.15 ppb. For more than half of the VOCs, the NH3 concentration falls below the LOQ for all or most measurement. This demonstrates a very low interference from the investigated VOCs.
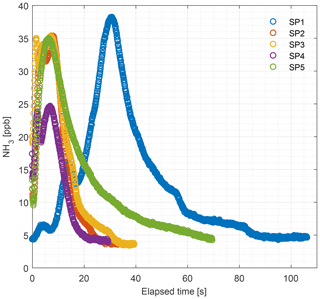
Figure 10Levels of NH3 concentration in the 2-week-old external particulate filters (PTFE, 0.20 µm pore size) measured by the CRDS in the laboratory. Filters collected from the field were installed before each sampling port. SP denotes sampling point.
The results of this study clearly demonstrate the advantage of CRDS with only few and small interferences, whereas the performance of the photoacoustic analyzers under similar circumstances studied by Liu et al. (2019) showed much more interference from non-NH3 species.
Our tests of the Picarro CRDS showed great linearity during both laboratory and fieldwork. The response times with respect to concentration changes were sufficiently low to measure temporal variations in NH3 concentrations in livestock emissions. Examinations of external particulate filters lead to no clear recommendations for filter material, but all filters gave acceptable response times and only small amounts of NH3 adsorption compared to background levels. Potential interferences were tested for 10 VOCs in known concentrations and the compounds gave negligible interference on CRDS NH3 measurements.
The data in the study are available upon request to the corresponding author (af@eng.au.dk).
Table A1Measured concentrations of NH3, CO2 and CH4 in laboratory zero air (CV denotes coefficient of variation).
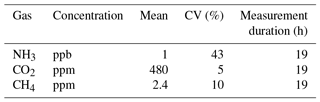
Table A2The rise (1∕e response time) and fall (1∕e response time) times (s) of the CRDS analyzer for measurements of NH3 concentrations (0.203 and 10.01 ppm) with or without external inlet particulate filters during laboratory testing. For filters detail see Table 1.
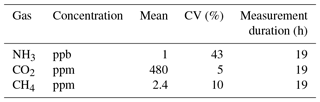
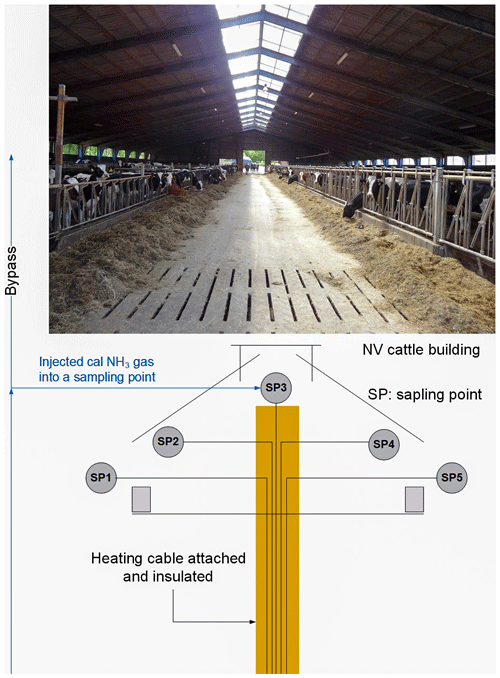
Figure A1Schematic of the sampling point inside the cattle building. SP1 and SP5 were placed outside for background measurement at 2.5 m height. SP2 and SP4 were on the walls at 2.5 m height. SP3 was placed below the ridge at 9 m height. The lines were approximately 5, 15, 35, 45 and 50 m for SP1, SP2, SP3, SP4 and SP5, respectively.
JK and AC performed the experiments, analysis and drafted the initial manuscript. The work was conceptualized and validated by AF, AC and JK with input from APA on the methodology. All authors have contributed and edited the paper during the review.
The authors declare that they have no conflict of interest.
The authors thank laboratory technician Heidi Grønbæk Christiansen and technician Peter Storegård Nielsen for their valuable help during the experimental part of the study. The authors thank the Danish Milk Levy Foundation for funding this research.
This research has been supported by the Danish Milk Levy Foundation.
This paper was edited by Folkert Boersma and reviewed by two anonymous referees.
Aneja, V. P., Roelle, P. A., Murray, G. C., Southerland, J., Erisman, J. W., Fowler, D., Asman, W. A. H., and Patni, N.: Atmospheric nitrogen compounds II: emissions, transport, transformation, deposition and assessment, Atmos. Environ., 35, 1903–1911, https://doi.org/10.1016/S1352-2310(00)00543-4, 2001.
Baek, B. H., Aneja, V. P., and Tong, Q.: Chemical coupling between ammonia, acid gases, and fine particles, Environ. Pollut., 129, 89–98, https://doi.org/10.1016/j.envpol.2003.09.022, 2004.
Bobrutzki, K. von, Braban, C. F., Famulari, D., Jones, S. K., Blackall, T., Smith, T. E. L., Blom, M., Coe, H., Gallagher, M., Ghalaieny, M., McGillen, M. R., Percival, C. J., Whitehead, J. D., Ellis, R., Murphy, J., Mohacsi, A., Pogany, A., Junninen, H., Rantanen, S., Sutton, M. A., and Nemitz, E.: Field inter-comparison of eleven atmospheric ammonia measurement techniques, Atmos. Meas. Tech., 3, 91–112, https://doi.org/10.5194/amt-3-91-2010, 2010.
Brown, P., Watts, P., Märk, T. D., and Mayhew, C. A.: Proton transfer reaction mass spectrometry investigations on the effects of reduced electric field and reagent ion internal energy on product ion branching ratios for a series of saturated alcohols, Int. J. Mass Spectrom., 294, 103–111, https://doi.org/10.1016/j.ijms.2010.05.028, 2010.
Copeland, N., Cape, J. N., and Heal, M. R.: Volatile organic compound emissions from Miscanthus and short rotation coppice willow bioenergy crops, Atmos. Environ., 60, 327–335, https://doi.org/10.1016/j.atmosenv.2012.06.065, 2012.
Feilberg, A., Liu, D., Adamsen, A. P. S., Hansen, M. J., and Jonassen, K. E. N.: Odorant Emissions from Intensive Pig Production Measured by Online Proton-Transfer-Reaction Mass Spectrometry, Environ. Sci. Technol., 44, 5894–5900, https://doi.org/10.1021/es100483s, 2010.
Gordon, I. E., Rothman, L. S., Hill, C., Kochanov, R. V, Tan, Y., Bernath, P. F., Birk, M., Boudon, V., Campargue, A., Chance, K. V, Drouin, B. J., Flaud, J., Gamache, R. R., Hodges, J. T., Jacquemart, D., Perevalov, V. I., Perrin, A., Shine, K. P., Smith, M. H., Tennyson, J., Toon, G. C., Tran, H., Tyuterev, V. G., Barbe, A., Császár, A. G., Devi, V. M., Furtenbacher, T., Harrison, J. J., Hartmann, J., Jolly, A., Johnson, T. J., Karman, T., Kleiner, I., Kyuberis, A. A., Loos, J., Lyulin, O. M., Massie, S. T., Mikhailenko, S. N., Moazzen-ahmadi, N., Müller, H. S. P., Naumenko, O. V, Nikitin, A. V, Polyansky, O. L., Auwera, J. Vander, Wagner, G., Wilzewski, J., Wcis, P., Yu, S., and Zak, E. J.: Journal of Quantitative Spectroscopy & Radiative Transfer The HITRAN2016 molecular spectroscopic database, J. Quant. Spectrosc. Ra., 203, 3–69, https://doi.org/10.1016/j.jqsrt.2017.06.038, 2017.
Hafner, S. D., Howard, C., Muck, R. E., Franco, R. B., Montes, F., Green, P. G., Mitloehner, F., Trabue, S. L., and Rotz, C. A.: Emission of volatile organic compounds from silage: Compounds, sources, and implications, Atmos. Environ., 77, 827–839, https://doi.org/10.1016/j.atmosenv.2013.04.076, 2013.
Hansen, M. J., Liu, D., Guldberg, L. B., and Feilberg, A.: Application of proton-transfer-reaction mass spectrometry to the assessment of odorant removal in a biological air cleaner for pig production, J. Agr. Food Chem., 60, 2599–2606, https://doi.org/10.1021/jf300182c, 2012.
Hassouna, M., Robin, P., Charpiot, A., Edouard, N., and Méda, B.: Infrared photoacoustic spectroscopy in animal houses: Effect of non-compensated interferences on ammonia, nitrous oxide and methane air concentrations, Biosyst. Eng., 114, 318–326, https://doi.org/10.1016/j.biosystemseng.2012.12.011, 2013.
Heber, A. J., Ni, J.-Q., Lim, T. T., Tao, P.-C., Schmidt, A. M., Koziel, J. A., Beasley, D. B., Hoff, S. J., Nicolai, R. E., Jacobson, L. D., and Zhang, Y.: Quality Assured Measurements of Animal Building Emissions: Gas Concentrations, J. Air Waste Manage. Assoc., 56, 1472–1483, https://doi.org/10.1080/10473289.2006.10465680, 2006.
Huszár, H., Pogány, A., Bozóki, Z., Mohácsi, Á., Horváth, L., and Szabó, G.: Ammonia monitoring at ppb level using photoacoustic spectroscopy for environmental application, Sensors Actuat. B-Chem., 134, 1027–1033, https://doi.org/10.1016/j.snb.2008.05.013, 2008.
Koerkamp, P. W. G. G., Metz, J. H. M., Uenk, G. H., Phillips, V. R., Holden, M. R., Sneath, R. W., Short, J. L., White, R. P. P., Hartung, J., Seedorf, J., Schröder, M., Linkert, K. H., Pedersen, S., Takai, H., Johnsen, J. O., and Wathes, C. M.: Concentrations and Emissions of Ammonia in Livestock Buildings in Northern Europe, J. Agr. Eng. Res., 70, 79–95, https://doi.org/10.1006/jaer.1998.0275, 1998.
Liu, D., Rong, L., Kamp, J., Kong, X., Adamsen, A. P., Chowdhury, A., and Feilberg, A.: Photoacoustic measurement may significantly overestimate NH3 emissions from cattle houses due to VOC interferences, Atmos. Meas. Tech. Discuss., https://doi.org/10.5194/amt-2018-412, in review, 2019.
Maasikmets, M., Teinemaa, E., Kaasik, A., and Kimmel, V.: Measurement and analysis of ammonia, hydrogen sulphide and odour emissions from the cattle farming in Estonia, Biosyst. Eng., 139, 48–59, https://doi.org/10.1016/j.biosystemseng.2015.08.002, 2015.
Martin, N. A., Ferracci, V., Cassidy, N., and Hoffnagle, J. A.: The application of a cavity ring-down spectrometer to measurements of ambient ammonia using traceable primary standard gas mixtures, Appl. Phys. B, 122, 1–11, https://doi.org/10.1007/s00340-016-6486-9, 2016.
Ngwabie, N. M., Schade, G. W., Custer, T. G., Linke, S., and Hinz, T.: Abundances and Flux Estimates of Volatile Organic Compounds from a Dairy Cowshed in Germany, J. Environ. Qual., 37, 565–573, https://doi.org/10.2134/jeq2006.0417, 2008.
Ngwabie, N. M., Jeppsson, K. H., Nimmermark, S., Swensson, C., and Gustafsson, G.: Multi-location measurements of greenhouse gases and emission rates of methane and ammonia from a naturally-ventilated barn for dairy cows, Biosyst. Eng., 103, 68–77, https://doi.org/10.1016/j.biosystemseng.2009.02.004, 2009.
Ni, J.-Q. and Heber, A. J.: Sampling and Measurement of Ammonia at Animal Facilities, Adv. Agron., 98, 201–269, 2008.
Nielsen, O. K., Plejdrup, M. S., Winther, M., Mikkelsen, M. H., Nielsen, M., Gyldenkærne, S., Fauser, P., Albrektsen, R., Hjelgaard, K. H., Bruun, H. G., and Thomsen, M.: Annual Danish Informative Inventory Report to UNECE, Emission inventories from the base year of the protocols to year 2015, Aarhus University, DCE – Danish Centre for Environment and Energy, 2017.
Picarro, I.: NH3 Analyzer for Ambient Air Datasheet, archived by WebCite®, available at: http://www.webcitation.org/70JfzdB0u (last access: 20 June 2018), 2017.
Poissant, L., Pilote, M., Beauvais, C., Constant, P., and Zhang, H. H.: A year of continuous measurements of three atmospheric mercury species (GEM, RGM and Hg p) in southern Québec, Canada, Atmos. Environ., 39, 1275–1287, https://doi.org/10.1016/j.atmosenv.2004.11.007, 2005.
Rom, H. B. and Zhang, G. Q.: Time delay for aerial Ammonia concentration measurements in livestock buildings, Sensors, 10, 4634–4642, https://doi.org/10.3390/s100504634, 2010.
Rong, L., Liu, D., Pedersen, E. F., and Zhang, G.: Effect of climate parameters on air exchange rate and ammonia and methane emissions from a hybrid ventilated dairy cow building, Energy Build., 82, 632–643, https://doi.org/10.1016/j.enbuild.2014.07.089, 2014.
Rosenstock, T. S., Diaz-Pines, E., Zuazo, P., Jordan, G., Predotova, M., Mutuo, P., Abwanda, S., Thiong'o, M., Buerkert, A., Rufino, M. C., Kiese, R., Neufeldt, H., and Butterbach-Bahl, K.: Accuracy and precision of photoacoustic spectroscopy not guaranteed, Glob. Change Biol., 19, 3565–3567, https://doi.org/10.1111/gcb.12332, 2013.
Saha, C. K., Zhang, G., Kai, P., and Bjerg, B.: Effects of a partial pit ventilation system on indoor air quality and ammonia emission from a fattening pig room, Biosyst. Eng., 105, 279–287, https://doi.org/10.1016/j.biosystemseng.2009.11.006, 2010.
Shah, S. B., Grabow, G. L., and Westerman, P. W.: Ammonia Adsorption in Five Types of Flexible Tubing Materials, Am. Soc. Agr. Biol. Eng., 22, 919–923, 2006.
Shaw, S. L., Mitloehner, F. M., Jackson, W., Depeters, E. J., Fadel, J. G., Robinson, P. H., Holzinger, R., and Goldstein, A. H.: Volatile organic compound emissions from dairy cows and their waste as measured by proton-transfer-reaction mass spectrometry, Environ. Sci. Technol., 41, 1310–1316, https://doi.org/10.1021/es061475e, 2007.
Sheppard, L. J., Leith, I. D., Mizunuma, T., Neil Cape, J., Crossley, A., Leeson, S., Sutton, M. A., van Dijk, N., and Fowler, D.: Dry deposition of ammonia gas drives species change faster than wet deposition of ammonium ions: evidence from a long-term field manipulation, Glob. Change Biol., 17, 3589–3607, https://doi.org/10.1111/j.1365-2486.2011.02478.x, 2011.
Sintermann, J., Ammann, C., Kuhn, U., Spirig, C., Hirschberger, R., Gärtner, A., and Neftel, A.: Determination of field scale ammonia emissions for common slurry spreading practice with two independent methods, Atmos. Meas. Tech., 4, 1821–1840, https://doi.org/10.5194/amt-4-1821-2011, 2011.
Vaittinen, O., Metsälä, M., Persijn, S., Vainio, M., and Halonen, L.: Adsorption of ammonia on treated stainless steel and polymer surfaces, Appl. Phys. B, 115, 185–196, https://doi.org/10.1007/s00340-013-5590-3, 2014.
Wu, W., Zhang, G., and Kai, P.: Ammonia and methane emissions from two naturally ventilated dairy cattle buildings and the influence of climatic factors on ammonia emissions, Atmos. Environ., 61, 232–243, https://doi.org/10.1016/j.atmosenv.2012.07.050, 2012.
Yuan, B., Coggon, M. M., Koss, A. R., Warneke, C., Eilerman, S., Peischl, J., Aikin, K. C., Ryerson, T. B., and De Gouw, J. A.: Emissions of volatile organic compounds (VOCs) from concentrated animal feeding operations (CAFOs): Chemical compositions and separation of sources, Atmos. Chem. Phys., 17, 4945–4956, https://doi.org/10.5194/acp-17-4945-2017, 2017.
Zhang, G., Strøm, J. S., Li, B., Rom, H. B., Morsing, S., Dahl, P., and Wang, C.: Emission of ammonia and other contaminant gases from naturally ventilated dairy cattle buildings, Biosyst. Eng., 92, 355–364, https://doi.org/10.1016/j.biosystemseng.2005.08.002, 2005.
Zhao, Y., Pan, Y., Rutherford, J., and Mitloehner, F. M.: Estimation of the interference in Multi-Gas measurements using infrared photoacoustic analyzers, Atmosphere, 3, 246–265, https://doi.org/10.3390/atmos3020246, 2012.
Zhu, X., Burger, M., Doane, T. A., and Horwath, W. R.: Ammonia oxidation pathways and nitrifier denitrification are significant sources of N2O and NO under low oxygen availability, P. Natl. Acad. Sci. USA, 110, 6328–6333, 2013.
Zong, C., Feng, Y., Zhang, G., and Hansen, M. J.: Effects of different air inlets on indoor air quality and ammonia emission from two experimental fattening pig rooms with partial pit ventilation system – Summer condition, Biosyst. Eng., 122, 163–173, https://doi.org/10.1016/j.biosystemseng.2014.04.005, 2014.