the Creative Commons Attribution 4.0 License.
the Creative Commons Attribution 4.0 License.
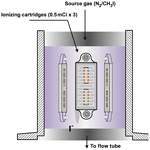
A low-activity ion source for measurement of atmospheric gases by chemical ionization mass spectrometry
Young Ro Lee
Yi Ji
David J. Tanner
Most I−-CIMSs (iodide chemical ionization mass spectrometers) for measurement of atmospheric trace gases utilize a radioactive ion source with an initial activity of 10 or 20 mCi of 210Po. In this work, we characterize a 210Po ion source with an initial activity of 1.5 mCi that can be easily constructed from commercially available components. The low level of radioactive activity of this source significantly reduces regulatory burden with storage and shipping relative to higher-activity sources. We compare the sensitivity of the low-activity source (LAS) to a standard 20 mCi source, as a function of carrier gas flow and flow tube pressure, for peroxyacetyl nitrate (PAN), formic acid (HCO2H), molecular chlorine (Cl2) and nitryl chloride (ClNO2), using an I−-CIMS. The LAS provides 2 to 5 times less sensitivity than that of the standard source even though the ratio of activity is approximately 13. However, detection limits of less than 2 pptv for the tested compounds are achieved for integration times on the order of a minute. The sensitivity of the LAS is less dependent on the magnitude of the carrier gas than a standard source. In addition, the LAS provides maximum sensitivity at relatively low carrier gas flows. Finally, we demonstrate that the LAS can be used to measure PAN in the remote atmosphere from an aircraft by showing data obtained on the NASA DC-8 during the Atmospheric Tomography (ATom) mission. In summary, the LAS may be an excellent substitute for a standard ion source in short-term field deployments.
- Article
(3956 KB) - Full-text XML
-
Supplement
(1048 KB) - BibTeX
- EndNote
Chemical ionization mass spectrometry (CIMS) is a versatile method for the measurement of atmospheric trace gases (Huey, 2007). CIMS has excellent sensitivity and a rapid time response (t<1 s) which allows the observation of compounds with high temporal resolution, which is particularly important for airborne measurements. There are a variety of different CIMS methods used to measure atmospheric species such as PTR-MS (proton-transfer mass spectrometry), atmospheric pressure CIMS commonly utilizing as a reagent ion, and reduced-pressure CIMS utilizing I− and other reagent ions such as CF3O−, acetate, and (Lindinger et al., 1998; de Gouw and Warneke, 2007; Eisele and Tanner, 1993; Huey et al., 1995, 1996; Crounse et al., 2006; Veres et al., 2008; Bertram et al., 2011; Slusher et al., 2001).
The defining characteristic of CIMS is ionization of a compound by a chemical reaction with a reagent ion. Reagent ions are produced by flowing precursor gases through an ion source. Typically, most I−-CIMSs flow dilute methyl iodide in nitrogen through a tube containing a 210Po metal foil which emits alpha particles. The alpha particles ionize the nitrogen, producing secondary electrons that can be thermalized and dissociatively attached by methyl iodide to form I−. PTR-MS instruments typically use a hollow cathode electrical discharge to produce H3O+ ions that can be introduced into a drift tube reactor (de Gouw et al., 2003; Inomata et al., 2006). Other common ion sources include X-ray, americium (alpha emitter) and corona discharges (Jost et al., 2003; Kürten et al., 2011; Zheng et al., 2015). The choice of an ion source for a specific application is influenced by many factors that include operating pressure, ion current, background contamination and regulatory environment. For example, radioactive ion sources are generally not used at operating pressures below 13 mbar (10 torr) as the path length of the emitted alpha particles is too large to generate sufficient ion current. In contrast, PTR-MS typically operates at ionization pressures below 1 torr, which is more compatible with a discharge source (Yuan et al., 2017).
The use of radioactive ion sources for CIMS has several significant advantages. These include stability, ease of use and reliability. Perhaps the most important feature is the efficiency of ion generation. A 20 mCi 210Po ion source produces 7.4×108 disintegrations per second. This dissipates less than 1 mW of power and can produce more than 107 reagent ions per second impacting an ion detector (Nah et al., 2018). Thus, high ion count rates are achieved while limiting the production of radicals that can react and produce molecules that interfere with measurement of atmospheric species.
There are significant disadvantages to radioactive ion sources as well. Polonium sources need to be replaced regularly, due to a half-life of 138 d, at some expense. More importantly polonium is highly toxic, and most 210Po sources require hazmat shipping and a significant degree of institutional oversight. For example, standard radioactive sources used in CIMS are commercial antistatic devices, P-2021 (10 mCi) and P-2031 (20 mCi), manufactured by NRD. Both of these sources require hazmat shipping if returned to the manufacturer on the timescale of months. The activity level of these sources may constrain their use in field locations for which permissions to transport or use radioactive source may not be feasible (49 CFR 173.425, 2011, Table 4). In addition, while these commercially available devices are designed to be used in evacuated systems, they may require additional preparation to ensure a good vacuum seal. For example, we have found that a significant fraction of the 20 mCi ionizers have vacuum leaks (e.g., leaks on the screws under the label are common).
One problem that we have repeatedly encountered at remote field sites or at aircraft facilities is a lack of ability to store the radioactive sources, which necessitates shipping the source back to the manufacturer immediately after the field observations are completed. However, most field campaigns are on the order of a month or two in duration, which necessitates hazmat shipping of the standard sources. The goal of this work is to develop a source with lower activity that we can easily ship back to the manufacturer after field use. In this paper, we describe a 210Po ion source with an initial activity of 1.5 mCi using standard antistatic products, commonly employed in mass balances, mounted in commercially available vacuum fittings, which can be easily interchanged with traditional radioactive ion sources. This initial level of activity for the LAS, below the maximum activity limit for excepted package of 200 MBq, does not require specific packaging, labeling and documentation except for the UN identification number (i.e., UN2911) marked on the outside of the package and included in the packing list (49 CFR 173.410; 49 CFR 173.425, 2011, Table 4). The 500 µCi static eliminator cartridges are a generally licensed device. However, the degree of regulation is specific to the institutions and states in which they are deployed. We compare the sensitivity of this source to a standard 20 mCi source for CIMS measurements of peroxyacetyl nitrate (PAN), molecular chlorine (Cl2), nitryl chloride (ClNO2) and formic acid (HCO2H). We also show the performance of the LAS for airborne measurement of PAN from the NASA DC-8 research aircraft during the Atmospheric Tomography (ATom) mission.
2.1 Low-activity ion source
A drawing and photograph of the LAS are shown in Fig. 1. The LAS contains three commercial antistatic ionizing cartridges (2U500, NRD), housed in a standard QF-40 nipple. Each cartridge has an initial activity of 500 µCi equivalent to 1.85×107 disintegrations per second and is equipped with a protective grid to prevent physically touching of the polonium foil (Fig. 1b). The cartridges, with a total activity of 1.5 mCi (55.5 MBq), were mounted on a thin (∼0.1 mm) stainless-steel foil that is 6 cm wide and 10 cm high as shown in Fig. 1a. The foil with the cartridges were folded to fit in a QF-40 nipple. The QF-40 stainless-steel nipple containing ionizing cartridges was connected to a flow tube reactor (QF-40 stainless-steel tee). The opposite opening of the nipple was connected to a manifold that enables delivery of a flow of dilute methyl iodide (CH3I) in nitrogen.
2.2 Laboratory configuration for TD-CIMS PAN measurement technique
Characterization of the LAS is performed using the TD-CIMS technique for PAN measurement (Slusher et al., 2004; Zheng et al., 2011). The CIMS instrument used herein is very similar to that described by Kim et al. (2007) and Chen et al. (2016). As shown in Fig. 2, the CIMS consists of the following differently pumped regions: a flow tube, a collisional dissociation chamber (CDC), an octopole ion guide and a quadrupole mass filter with an ion detector. Ambient air is sampled through an inlet constructed from 1.27 cm outer diameter and 0.95 cm inner diameter FEP Teflon tubing at a constant flow rate of approximately 2.7 standard liters per minute (slpm). Background measurements were performed regularly by drawing ambient air through a QF 40 nipple filled with stainless-steel wool heated to 150 ∘C, which effectively destroys the thermally generated radicals (Flocke et al., 2005; Zheng et al., 2011). A 29.6 cm section of the Teflon tubing in front of the flow tube orifice is heated to an external temperature of approximately 180 ∘C to thermally dissociate PAN (Reaction R1). It should be noted that longer length and higher temperature than typical operation conditions for PAN measurements are used to achieve full thermal dissociation of PAN within the residence time in the heated inlet. Additional details are provided in Fig. S1 in the Supplement.
The thermal dissociation (TD) region is evacuated into the CIMS and a small scroll pump (Varian IDP3; 60 L min−1). The TD region was maintained at a constant pressure of 100 torr by the combination of an upstream metering valve and a commercial pressure controller (MKS 640) mounted downstream of the TD before the small scroll pump. Reduced pressure in the thermal dissociation region minimizes negative interferences (e.g., loss of sensitivity) due to NO, NO2 and radical–radical reactions. The impact of ambient NO and NO2 on sensitivity was minimal in these experiments as shown in Fig. S1c and d. The flow rate before the pressure controller was held constant at 2.7 slpm so that a constant dilution of the calibration standard and thermal dissociation conditions was maintained. The sample mass flow into the low-pressure flow tube is controlled by an automatic variable orifice (AVO). The area of a triangular opening in the AVO varies by a microprocessor-controlled stepping motor. The AVO is used in these experiments to maintain a constant total mass flow into the flow tube as the ion source flow is varied. This feature has been utilized in Chen et al. (2016) to maintain a constant flow tube pressure over the vertical range of aircraft sampling. An example of the gas flows at a flow tube pressure of 40 torr is shown in Fig. S1a.
The experiments were carried out using two different scroll pumps (Edwards nXDS 6i or Varian Triscroll™ 300) with corresponding pumping speeds of 105 and 240 L min−1. This allowed us to evaluate the use of the LAS under conditions typical of both airborne and ground-based operation. We commonly use a large scroll pump for ground-based measurements but are often required to use a smaller scroll pump due to power and weight limitations encountered on aircraft. Flow tube pressures higher than 20 torr were achieved by placing a restrictive orifice between the flow tube and the scroll pump. The total flows sampled into the scroll pumps were 2.7 and 6.5 slpm, respectively. Iodide reagent ions (I−) are produced by passing dilute methyl iodide in nitrogen through a tube containing 210Po (1.5 or 20 mCi). Ion–molecule reactions occur over the length of the flow tube (Reactions R2–R3), and the resulting product ions are transmitted into a collisional dissociation chamber (CDC) evacuated by a molecular drag pump (Alcatel MDP 5011). Weakly bound cluster ions are dissociated into core ions in the CDC, e.g., dissociates into RC(O)O−. The ions are guided by octopole to the quadrupole filter for mass selection and then detected by an electron multiplier. PAN is quantified as acetate anion, 59 m∕z or 61 m∕z , in the TD-CIMS.
2.3 Laboratory configuration for iodide-adduct CIMS
In addition to the characterization of the LAS using the TD-CIMS technique, similar experiments are performed to demonstrate the capabilities of the LAS using iodide-adduct formation for the detection of formic acid, Cl2 and ClNO2. This tests the LAS for a more generally used chemical ionization method (e.g., Le Breton et al., 2012; Lee et al., 2014; Osthoff et al., 2008; Thornton et al., 2010; Mielke et al., 2011). The configuration of the CIMS system used in this work is very similar to that described in the previous section and is shown in Fig. S2. For this experiment, the flow tube was humidified by a constant amount of water vapor by passing a flow of 10 ccm of pure N2 through a water bubbler kept in an ice bath in order to mitigate variations in instrument sensitivity affected by ambient humidity.
Calibration sources
The isotopically labeled PAN calibration standard was generated from a photolytic source similar to that described by Warneck and Zerbach (1992). The output of the photolytic source was periodically added to PAN-free air, downstream of the heated metal tubing, which limits potential interference from ambient peroxyacetic acid (Phillips et al., 2013).
A permeation tube (KIN-TEK Laboratories, Inc.) was used as a calibration standard for formic acid. The permeation rate of the formic acid permeation tube was quantified by passing the output of the permeation tube in a flow of 50 sccm of N2 through DI water. The resulting water solution of formic acid was analyzed using ion chromatography (Metrohm 761 compact ICs). A permeation tube (KIN-TEK Laboratories, Inc.) was used as a calibration standard for Cl2. To determine the emission rate of the Cl2 permeation tube, the output was measured using a method described in detail by Kazantseva et al. (2002) and Chen et al. (2016).
The ClNO2 standard was generated by passing a humidified flow of Cl2 in N2 through a bed of sodium nitrite (NaNO2) via the following reaction:
The resulting ClNO2 was quantified by passing it through a heated quartz tube (150–230 ∘C). NO2 was generated via thermal dissociation of ClNO2 and measured by a CAPS (cavity attenuated phase sift) NO2 monitor (Thaler et al., 2011; Kebabian et al., 2008). The sensitivity for ClNO2 was acquired by measuring the increase in the NO2 concentration simultaneously with the decrease in ClNO2 signal in CIMS.
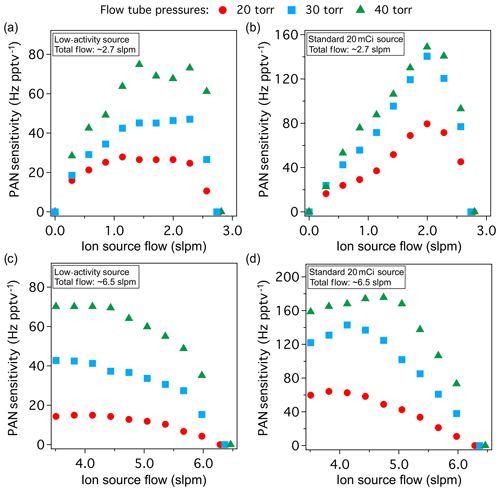
Figure 3The sensitivity (Hz pptv−1) of the LAS and a standard source for PAN (m∕z 61) at different flow tube pressures and total flows, plotted as a function of ion source flow. The ages of the LAS and the standard source used here are approximately 2 and 4 months old, respectively. (a) PAN sensitivity using the LAS and (b) the standard source at total flow of ∼2.7 slpm. (c) PAN sensitivity using the LAS and (d) the standard source at total flow of ∼6.5 slpm. Red circles, cyan squares and green triangles correspond to flow tube pressures of 20, 30 and 40 torr, respectively.
3.1 Performance of LAS for the CIMS measurement of PAN
Performance of the LAS is compared to a standard 20 mCi radioactivity source by examining the detection sensitivity and the background signal level. Figure 3a and b show the sensitivity of both sources to 13C-PAN as a function of ion source flow at total flow of roughly 2.7 and 6.5 slpm, respectively. In general, the sensitivity of the LAS is approximately 2 to 4 times lower than that of the standard source, whereas the activity ratio is roughly 13. Thus, it is likely that the initial activity of the LAS can result in higher sensitivity than the standard source aged to an equivalent activity level (∼18 months), where the time dependence of sensitivity for both sources is discussed in Sect. 3.4. Both sources at two different total flows give sensitivities on the order of tens of Hz pptv−1. The ion signals during background measurements (i.e., air through the heated stainless-steel wool) range from tens to thousands hertz for both sources, where the LAS shows generally lower levels by a factor of ∼2. The detection limits (LODs) for PAN using the LAS were comparable to that of the standard source, resulting in less than 2 pptv for a 2 min integration (for a 23 % duty cycle at m∕z 59), where LOD were estimated as 3 times the standard deviation values (3σ) of the background level. Maximum sensitivity was observed at flow tube pressures of 40 torr for both sources and total flows. We could undoubtedly gain more sensitivity at flow tube pressures higher than 40 torr. However, we have found that higher pressures significantly enhance the risks of interference from unwanted secondary chemistry (Chen et al., 2016). For experiments at higher total flow, the sensitivities of both sources were similar to those at lower total flow but less sensitive to changes in ion source flow (Fig. 3c and d). The tests do show that the LAS is more sensitive to pressure than the standard source. In contrast, the sensitivity of the LAS for PAN is significantly less dependent on the ion source carrier gas flow compared to the standard source (Fig. 3a). In particular, the LAS provides near maximum sensitivity at ion source flows a little greater than 1 slpm as illustrated in Fig. 3a. Thus, a low-activity source may be preferable in some applications in which reaching maximum sensitivity is not necessary (e.g., high ambient mixing ratios of targeting species) and the availability of carrier gas is limited.
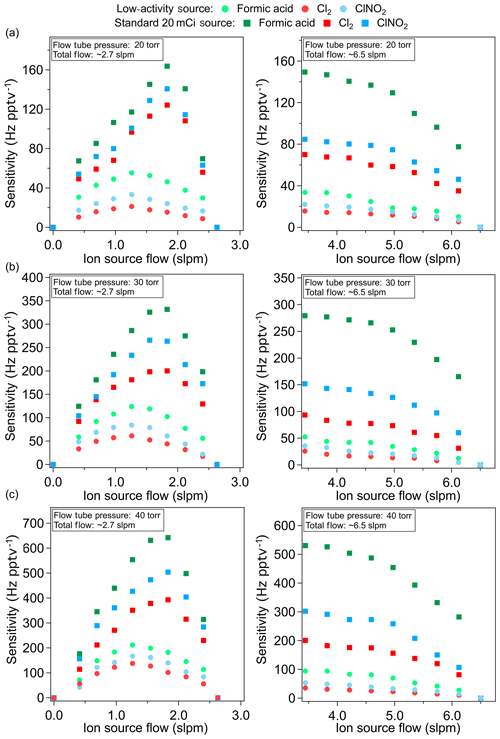
Figure 4Comparison of the sensitivity (Hz pptv−1) of the LAS and a standard source for formic acid (m∕z 179, green), Cl2 (m∕z 197, red) and ClNO2 (m∕z 208, cyan) plotted as a function of ion source flow (slpm). The age of the LAS and the standard source used here is approximately 2 and 4 months old, respectively. Panels (a)–(c) show the sensitivity at flow tube pressures of 20, 30 and 40 torr at a total flow of 2.7 slpm (left) and 6.5 slpm (right).
3.2 Performance of LAS for the CIMS measurement of formic acid, Cl2 and ClNO2
Figure 4 presents the sensitivity comparison for the detection of formic acid, Cl2 and ClNO2, where ion signals are monitored at m∕z 179, m∕z 197 and m∕z 208, respectively. The trends presented were generally consistent with the PAN measurements. LODs were 1, 1 and 0.5 pptv, respectively, with an integration time of 1 min. The sensitivity of the LAS is approximately 3 to 5 times lower than that of the standard source. Maximum sensitivity for both sources was observed at a flow tube pressure of 40 torr for all three species. Similar to the measurements of PAN, the ratio of the LAS sensitivity to that of the standard source is greater at lower flows.
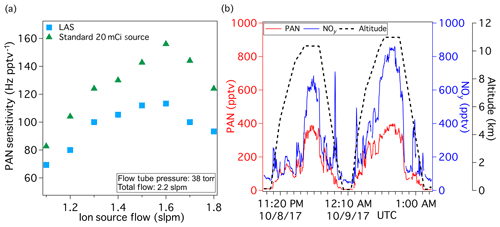
Figure 5The performance of the LAS for PAN measurement during the ATom field mission. Both sources are less than 1 month old. (a) The sensitivity comparison of the LAS and a standard source for the ATom instrument conditions. (b) DC-8 flight data obtained during intersects of a Southern Hemisphere plume. PAN (red trace) and NOy (blue trace) are plotted along with the pressure altitudes (black trace).
3.3 Application to studies of the ambient atmosphere
The performance of the LAS source is illustrated in airborne observations of PAN during the Atmospheric Tomography (ATom) field mission shown in Fig. 5. Global-scale sampling of the atmosphere, profiling continuously over a span of altitudes, was conducted by an extensive gas and aerosol payload on board of the NASA DC-8 research aircraft. Figure 5a illustrates the performance of the low-radioactivity source for the ATom instrument conditions along with the sensitivity values using a standard source. Figure 5b demonstrates flight data collected within a Southern Hemisphere biomass burning plume near New Zealand on 8 October 2017 (ATom-3 science flight #5), where gaseous reactive nitrogen (NOy) is dominated by PAN. Figure S3 shows the flight path colored by the mixing ratio of PAN. Inside the plume intersects, the clear variation in PAN signal – CH3C(O)O−; 59 amu – is observed with a good signal to background noise ratio. Outside of the plume, PAN signal decreased simultaneously with the observed NOy mixing ratios. Clearly, the LAS can provide sensitive, fast time response detection of PAN from an aircraft platform.
3.4 Time dependence of sensitivity
As 210Po ion sources age and lose activity the reagent signal decreases as well. In our experience, with a 20 mCi source you lose sensitivity at less than the decay rate of the 210Po. We typically observe a loss of sensitivity of about a factor of 2 in sensitivity after approximately 1 year. In order to estimate the time response of the sensitivity of the LAS we compared a fresh LAS to that of one that was decayed by approximately one 210Po half-life ( d). The sensitivity of the LAS (Fig. S4) was found to decay about a factor of 2 after one half-life. This indicates that the sensitivity of the LAS will decay faster than that of a standard source.
The relative sensitivity of the LAS and the standard 20 mCi source is not in proportion to their activity ratios. The ratio of LAS sensitivity to activity is clearly higher than for a standard source. A likely explanation for much of this difference is the geometry of the sources. The LAS contains three cartridges mounted on a stainless-steel foil place in a QF40 nipple. This gives an effective inner diameter of the ion source of ∼3 cm. The standard source, NRD P-2031, has a more compact geometry with NPT fittings on each end and an effective inner diameter of ∼1 cm. The different inner diameters impact ion source flow characteristics such as residence time and ion loss rates to the wall. For a given flow rate the larger ID LAS is expected to have a lower wall loss than the standard source. In addition, the larger ID of the LAS provides a longer path length for the emitted alpha particles. In short, the more open geometry of the LAS likely provides for more efficient transport of the generated ions. This is also in accord with the observation that the LAS signal decays at approximately the same rate as the activity of the 210Po.
A low-activity 210Po ion source with an initial activity of 1.5 mCi is a viable alternative to the higher-activity sources typically used for I−-CIMS. The sensitivity and detection limits of the low-activity ion source presented in this work are more than adequate to measure PAN, Cl2, ClNO2 and formic acid in most environments. In applications that require maximum sensitivity, and if the availability of carrier gas for the ion source flow is not limiting, a standard source is probably preferable. In field experiments that have limited or no radioactive storage the LAS may be a preferable option. The LAS may also be extended to other CIMS methods and reagent ions and will be a focus of our future ion source development.
All of the laboratory data used in this paper are available upon request from the corresponding author. The ATom data can be found at https://doi.org/10.3334/ORNLDAAC/1581 (Wofsy et al., 2018).
The supplement related to this article is available online at: https://doi.org/10.5194/amt-13-2473-2020-supplement.
YRL performed the TD-CIMS experiments and wrote the paper with assistance from LGH. YJ performed the iodide-adduct CIMS experiments. DJT assisted with all the experiments. All of the authors were involved in data interpretation and commented on the paper.
The authors declare that they have no conflict of interest.
The views expressed in this document are solely those of the authors and do not necessarily reflect those of the EPA. EPA does not endorse any products or commercial services mentioned in this publication and EPA Grant.
The NOy data were provided by Thomas Ryerson, Chelsea Thompson and Jeff Peischl. We thank the ATom science team and the NASA DC-8 flight crew for their support.
This research has been supported by the NASA (grant no. NNX15AT90G) and the EPA (grant no. R835882).
This paper was edited by Bin Yuan and reviewed by three anonymous referees.
49 CFR 173.421: Excepted packages for limited quantities of Class 7 (radioactive) materials, available at: https://www.govinfo.gov/content/pkg/CFR-2010-title49-vol2/pdf/CFR-2010-title49-vol2-sec173-421.pdf (last access: 13 May 2020), 2010.
49 CFR 173.425: Table of activity limits – excepted quantities and articles, available at: https://www.govinfo.gov/content/pkg/CFR-2011-title49-vol2/pdf/CFR-2011-title49-vol2-sec173-425.pdf (last access: 13 May 2020), 2011.
Bertram, T. H., Kimmel, J. R., Crisp, T. A., Ryder, O. S., Yatavelli, R. L. N., Thornton, J. A., Cubison, M. J., Gonin, M., and Worsnop, D. R.: A field-deployable, chemical ionization time-of-flight mass spectrometer, Atmos. Meas. Tech., 4, 1471–1479, https://doi.org/10.5194/amt-4-1471-2011, 2011.
Chen, D., Huey, L. G., Tanner, D. J., Salawitch, R. J., Anderson, D. C., Wales, P. A., Pan, L. L., Atlas, E. L., Hornbrook, R. S., Apel, E. C., Blake, N. J., Campos, T. L., Donets, V., Flocke, F. M., Hall, S. R., Hanisco, T. F., Hills, A. J., Honomichl, S. B., Jensen, J. B., Kaser, L., Montzka, D. D., Nicely, J. M., Reeves, J. M., Riemer, D. D., Schauffler, S. M., Ullmann, K., Weinheimer, A. J., and Wolfe, G. M.: Airborne measurements of BrO and the sum of HOBr and Br2 over the Tropical West Pacific from 1 to 15 km during the CONvective TRansport of Active Species in the Tropics (CONTRAST) experiment, J. Geophys. Res.-Atmos., 121, 12560–12578, https://doi.org/10.1002/2016JD025561, 2016.
Crounse, J. D., McKinney, K. A., Kwan, A. J., and Wennberg, P. O.: Measurement of Gas-Phase Hydroperoxides by Chemical Ionization Mass Spectrometry, Anal. Chem., 78, 6726–6732, https://doi.org/10.1021/ac0604235, 2006.
de Gouw, J. A. and Warneke, C.: Measurements of volatile organic compounds in the earth's atmosphere using proton-transfer-reaction mass spectrometry, Mass Spectrom. Rev., 26, 223–257, https://doi.org/10.1002/mas.20119, 2007.
de Gouw, J., Warneke, C., Karl, T., Eerdekens, G., van der Veen, C., and Fall, R.: Sensitivity and specificity of atmospheric trace gas detection by proton-transfer-reaction mass spectrometry, Int. J. Mass Spectrom., 223, 365–382, 2003.
Eisele, F. L. and Tanner, D. J.: Measurement of the gas phase concentration of H2SO4 and methane sulfonic acid and estimates of H2SO4 production and loss in the atmosphere, J. Geophys. Res.-Atmos., 98, 9001–9010, https://doi.org/10.1029/93JD00031, 1993.
Flocke, F. M., Weinheimer, A. J., Swanson, A. L., Roberts, J. M., Schmitt, R., and Shertz, S.: On the measurement of PANs by gas chromatography and electron capture detection, J. Atmos. Chem., 52, 19–43, https://doi.org/10.1007/s10874-005-6772-0, 2005.
Huey, L. G.: Measurement of trace atmospheric species by chemical ionization mass spectrometry: Speciation of reactive nitrogen and future directions, Mass Spectrom. Rev., 26, 166–184, https://doi.org/10.1002/mas.20118, 2007.
Huey, L. G., Hanson, D. R., and Howard, C. J.: Reactions of and I− with Atmospheric Trace Gases, J. Phys. Chem., 99, 5001–5008, 1995.
Huey, L. G., Villalta, P. W., Dunlea, E. J., Hanson, D. R., and Howard, C. J.: Reactions of CF3O− with Atmospheric Trace Gases, J. Phys. Chem., 100, 190–194, 1996.
Inomata, S., Tanimoto, H., Aoki, N., Hirokawa, J., and Sadanaga, Y.: A novel discharge source of hydronium ions for proton transfer reaction ionization: design, characterization, and performance, Rapid Commun. Mass Spectrom., 20, 1025–1029, https://doi.org/10.1002/rcm.2405, 2006.
Jost, C., Sprung, D., Kenntner, T., and Reiner, T.: Atmospheric pressure chemical ionization mass spectrometry for the detection of tropospheric trace gases: the influence of clustering on sensitivity and precision, Int. J. Mass Spectrom., 223, 771–782, https://doi.org/10.1016/S1387-3806(02)00963-6, 2003.
Kazantseva, N. N., Ernepesova, A., Khodjamamedov, A., Geldyev, O. A., and Krumgalz, B. S.: Spectrophotometric analysis of iodide oxidation by chlorine in highly mineralized solutions, Anal. Chim. Acta., 456, 105–119, 2002.
Kebabian, P. L., Wood, E. C., Herndon, S. C., and Freedman, A.: A Practical Alternative to Chemiluminescence-Based Detection of Nitrogen Dioxide: Cavity Attenuated Phase Shift Spectroscopy, Environ. Sci. Technol., 42, 6040–6045, https://doi.org/10.1021/ac048715y, 2008.
Kim, S., Huey, L. G., Stickel, R. E., Tanner, D. J., Crawford, J. H., Olson, J. R., Chen, G., Brune, W. H., Ren, X., Lesher, R., Wooldridge, P. J., Bertram, T. H., Perring, A., Cohen, R. C., Lefer, B. L., Shetter, R. E., Avery, M., Diskin, G., and Sokolik, I.: Measurement of HO2NO2 in the free troposphere during the intercontinental chemical transport experiment – North America 2004, J. Geophys. Res.-Atmos., 112, D12S01, https://doi.org/10.1029/2006jd007676, 2007.
Kürten, A., Rondo, L., Ehrhart, S., and Curtius, J.: Performance of a corona ion source for measurement of sulfuric acid by chemical ionization mass spectrometry, Atmos. Meas. Tech., 4, 437–443, https://doi.org/10.5194/amt-4-437-2011, 2011.
Le Breton, M., McGillen, M. R., Muller, J. B. A., Bacak, A., Shallcross, D. E., Xiao, P., Huey, L. G., Tanner, D., Coe, H., and Percival, C. J.: Airborne observations of formic acid using a chemical ionization mass spectrometer, Atmos. Meas. Tech., 5, 3029–3039, https://doi.org/10.5194/amt-5-3029-2012, 2012.
Lee, B. H., Lopez-Hilfiker, F. D., Mohr, C., Kurten, T., Worsnop, D. R., and Thornton, J. A.: An Iodide-Adduct High-Resolution Time-of-Flight Chemical-Ionization Mass Spectrometer: Application to Atmospheric Inorganic and Organic Compounds, Environ. Sci. Technol., 48, 6309–6317, https://doi.org/10.1021/es500362a, 2014.
Lindinger, W., Hansel, A., and Jordan, A.: On-line monitoring of volatile organic compounds at pptv levels by means of proton-transfer reaction mass spectrometry (PTR-MS) – Medical applications, food control and environmental research, Int. J. Mass Spectrom. Ion Processes, 173, 191–241, https://doi.org/10.1016/S0168-1176(97)00281-4, 1998.
Mielke, L. H., Furgeson, A., and Osthoff, H. D.: Observation of CINO2 in a mid-continental urban environment, Environ. Sci. Technol., 45, 8889–8896, https://doi.org/10.1021/es201955u, 2011.
Nah, T., Ji, Y., Tanner, D. J., Guo, H., Sullivan, A. P., Ng, N. L., Weber, R. J., and Huey, L. G.: Real-time measurements of gas-phase organic acids using chemical ionization mass spectrometry, Atmos. Meas. Tech., 11, 5087–5104, https://doi.org/10.5194/amt-11-5087-2018, 2018.
Osthoff, H. D., Roberts, J. M., Ravishankara, A. R.,Williams, E. J., Lerner, B. M., Sommariva, R., Bates, T. S., Coffman, D., Quinn, P. K., Dibb, J. E., Stark, H., Burkholder, J. B., Talukdar, R. K., Meagher, J., Fehsenfeld, F. C., and Brown, S. S.: High levels of nitryl chloride in the polluted subtropical marine boundary layer, Nat. Geosci., 1, 324–328, 2008.
Phillips, G. J., Pouvesle, N., Thieser, J., Schuster, G., Axinte, R., Fischer, H., Williams, J., Lelieveld, J., and Crowley, J. N.: Peroxyacetyl nitrate (PAN) and peroxyacetic acid (PAA) measurements by iodide chemical ionisation mass spectrometry: first analysis of results in the boreal forest and implications for the measurement of PAN fluxes, Atmos. Chem. Phys., 13, 1129–1139, https://doi.org/10.5194/acp-13-1129-2013, 2013.
Slusher, D. L., Pitteri, S. J., Haman, B. J., Tanner, D. J., and Huey, L. G.: A chemical ionization technique for measurement of pernitric acid in the upper troposphere and the polar boundary layer, Geophys. Res. Lett., 28, 3875–3878, https://doi.org/10.1029/2001gl013443, 2001.
Slusher, D. L., Huey, L. G., Tanner, D. J., Flocke, F. M., and Roberts, J. M.: A thermal dissociation-chemical ionization mass spectrometry (TD-CIMS) technique for the simultaneous measurement of peroxyacyl nitrates and dinitrogen pentoxide, J. Geophys. Res.-Atmos., 109, D19315, https://doi.org/10.1029/2004JD004670, 2004.
Thaler, R. D., Mielke, L. H., and Osthoff, H. D.: Quantification of nitryl chloride at part per trillion mixing ratios by thermal dissociation cavity ring-down spectroscopy, Anal. Chem., 83, 2761–2766, https://doi.org/10.1021/ac200055z, 2011.
Thornton, J. A., Kercher, J. P., Riedel, T. P., Wagner, N. L., Cozic, J., Holloway, J. S., Dube, W. P., Wolfe, G. M., Quinn, P. K., Middlebrook, A. M., Alexander, B., and Brown, S. S.: A large atomic chlorine source inferred from midcontinental reactive nitrogen chemistry, Nature, 464, 271–274, https://doi.org/10.1038/nature08905, 2010.
Veres, P., Roberts, J. M., Warneke, C., Welsh-Bon, D., Zahniser, M., Herndon, S., Fall, R., and de Gouw, J.: Development of negative-ion proton-transfer chemical-ionization mass spectrometry (NI-PT-CIMS) for the measurement of gas-phase organic acids in the atmosphere, Int. J. Mass Spectrom., 274, 48–55, https://doi.org/10.1016/j.ijms.2008.04.032, 2008.
Warneck, P. and Zerbach, T.: Synthesis of peroxyacetyl nitrate in air by acetone photolysis, Environ. Sci. Technol., 26, 74–79, 1992.
Wofsy, S. C., Afshar, S., Allen, H. M., Apel, E. C., Asher, E. C., Barletta, B., Bent, J., Bian, H., Biggs, B. C., Blake, D. R., Blake, N., Bourgeois, I., Brock, C. A., Brune, W. H., Budney, J. W., Bui, T. P., Butler, A., Campuzano-Jost, P., Chang, C. S., Chin, M., Commane, R., Correa, G., Crounse, J. D., Cullis, P. D., Daube, B. C., Day, D. A., Dean-Day, J. M., Dibb, J. E., DiGangi, J. P., Diskin, G. S., Dollner, M., Elkins, J. W., Erdesz, F., Fiore, A. M., Flynn, C. M., Froyd, K. D., Gesler, D. W., Hall, S. R., Hanisco, T. F., Hannun, R. A., Hills, A. J., Hintsa, E. J., Hoffman, A., Hornbrook, R. S., Huey, L. G., Hughes, S., Jimenez, J. L., Johnson, B. J., Katich, J. M., Keeling, R. F., Kim, M. J., Kupc, A., Lait, L. R., Lamarque, J.-F., Liu, J., McKain, K., Mclaughlin, R. J., Meinardi, S., Miller, D. O., Montzka, S. A., Moore, F. L., Morgan, E. J., Murphy, D. M., Murray, L. T., Nault, B. A., Neuman, J. A., Newman, P. A., Nicely, J. M., Pan, X., Paplawsky, W., Peischl, J., Prather, M. J., Price, D. J., Ray, E., Reeves, J. M., Richardson, M., Rollins, A. W., Rosenlof, K. H., Ryerson, T. B., Scheuer, E., Schill, G. P., Schroder, J. C., Schwarz, J. P., Clair, J. M. St., Steenrod, S. D., Stephens, B. B., Strode, S. A., Sweeney, C., Tanner, D., Teng, A. P., Thames, A. B., Thompson, C. R., Ullmann, K., Veres, P. R., Vieznor, N., Wagner, N. L., Watt, A., Weber, R., Weinzierl, B., Wennberg, P. O., Williamson, C. J., Wilson, J. C., Wolfe, G. M., Woods, C. T., and Zeng, L. H.: ATom: Merged Atmospheric Chemistry, Trace Gases, and Aerosols, ORNL DAAC, Oak Ridge, Tennessee, USA, https://doi.org/10.3334/ORNLDAAC/1581, 2018.
Yuan, B., Koss, A. R., Warneke, C., Coggon, M., Sekimoto, K., and de Gouw, J. A.: Proton-Transfer-Reaction Mass Spectrometry, Applications in Atmospheric Sciences, Chem. Rev., 117, 13187–13229, https://doi.org/10.1021/acs.chemrev.7b00325, 2017.
Zheng, J., Yang, D. S., Ma, Y., Chen, M. D., Cheng, J., Li, S. Z., and Wang, M.: Development of a new corona discharge based ion source for high resolution time-of-flight chemical ionization mass spectrometer to measure gaseous H2SO4 and aerosol sulfate, Atmos. Environ., 119, 167–173, https://doi.org/10.1016/j.atmosenv.2015.08.028, 2015.
Zheng, W., Flocke, F. M., Tyndall, G. S., Swanson, A., Orlando, J. J., Roberts, J. M., Huey, L. G., and Tanner, D. J.: Characterization of a thermal decomposition chemical ionization mass spectrometer for the measurement of peroxy acyl nitrates (PANs) in the atmosphere, Atmos. Chem. Phys., 11, 6529–6547, https://doi.org/10.5194/acp-11-6529-2011, 2011.