the Creative Commons Attribution 4.0 License.
the Creative Commons Attribution 4.0 License.
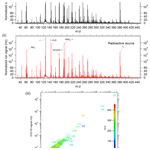
A vacuum ultraviolet ion source (VUV-IS) for iodide–chemical ionization mass spectrometry: a substitute for radioactive ion sources
Yi Ji
David J. Tanner
Young Ro Lee
Patrick R. Veres
J. Andrew Neuman
Yuhang Wang
Xinming Wang
A new ion source (IS) utilizing vacuum ultraviolet (VUV) light is developed and characterized for use with iodide–chemical ionization mass spectrometers (I−-CIMS). The VUV-IS utilizes a compact krypton lamp that emits light at two wavelengths corresponding to energies of ∼10.030 and 10.641 eV. The VUV light photoionizes either methyl iodide (ionization potential, IP = 9.54 ± 0.02 eV) or benzene (IP = 9.24378 ± 0.00007 eV) to form cations and photoelectrons. The electrons react with methyl iodide to form I−, which serves as the reagent ion for the CIMS. The VUV-IS is characterized by measuring the sensitivity of a quadrupole CIMS (Q-CIMS) to formic acid, molecular chlorine, and nitryl chloride under a variety of flow and pressure conditions. The sensitivity of the Q-CIMS, with the VUV-IS, reached up to ∼700 Hz pptv−1, with detection limits of less than 1 pptv for a 1 min integration period. The reliability of the Q-CIMS with a VUV-IS is demonstrated with data from a month-long ground-based field campaign. The VUV-IS is further tested by operation on a high-resolution time-of-flight CIMS (TOF-CIMS). Sensitivities greater than 25 Hz pptv−1 were obtained for formic acid and molecular chlorine, which were similar to that obtained with a radioactive source. In addition, the mass spectra from sampling ambient air was cleaner with the VUV-IS on the TOF-CIMS compared to measurements using a radioactive source. These results demonstrate that the VUV lamp is a viable substitute for radioactive ion sources on I−-CIMS systems for most applications. In addition, initial tests demonstrate that the VUV-IS can be extended to other reagent ions by the use of VUV absorbers with low IPs to serve as a source of photoelectrons for high IP electron attachers, such as .
- Article
(1615 KB) - Full-text XML
-
Supplement
(619 KB) - BibTeX
- EndNote
Chemical ionization mass spectrometry (CIMS) has been widely used as a powerful tool to measure various atmospheric compounds with high sensitivity and fast time response. CIMS measurements are based on selective ionization of compounds in air by reagent ions via ion molecule reactions. CIMS using the iodide anion (I−) and its water clusters as reagent ions (I−-CIMS) has been widely used in the measurements of many atmospheric trace gases, e.g., organic and inorganic acids (hydrogen chloride, HCl; nitric acid, HNO3; formic acid, HCOOH; etc.), halogens (bromine oxide, BrO; nitryl chloride, ClNO2; etc.), and peroxycarboxylic nitric anhydrides (PANs) (Slusher et al., 2004; Huey, 2007; Phillips et al., 2013; Lee et al., 2014; Liao et al., 2014; Neuman et al., 2016; Liu et al., 2017; Priestley et al., 2018; Bertram et al., 2011; Thornton et al., 2010; Osthoff et al., 2008).
Typically, I−-CIMS systems use a radioactive isotope, usually 210Po, as an ion source. 210Po emits α particles (with an energy of ∼5 MeV) that directly ionize the carrier gas in the ion source to produce secondary electrons. The secondary electrons are thermalized by collisions and react with methyl iodide (CH3I) to form I− by dissociative electron attachment. The use of radioactive ion sources with the I−-CIMS system is well established and has several important advantages. For example, radioactive sources are exceedingly reliable and easy to use, as they require no external power. Radioactive sources often produce relatively clean mass spectra with few interfering masses. However, radioactive sources have several disadvantages as well. 210Po is toxic and is highly regulated, which often makes it difficult to transport, store, and use in remote locations. We have recently developed a lower activity 210Po ion source that is subject to fewer regulatory restrictions (Lee et al., 2020). However, there remain applications where the use of any radioactivity is very difficult or prohibited. A more subtle disadvantage is that radioactive ion sources emit continuously, which can lead to the build-up of interfering species. For these reasons, it is desirable to find a nonradioactive alternative to efficiently generate I− and other reagent ions. Electrical discharges and x-ray ion sources have been used as ion sources in atmospheric pressure chemical ionization mass spectrometers (AP-CIMS) (Jost et al., 2003; Skalny et al., 2007; Kurten et al., 2011; Wang et al., 2017). However, they have not been commonly employed with I−-CIMS systems perhaps due to limited sensitivity and the generation of interfering ions. Recently Eger et al. (2019) developed a promising ion source using a radio frequency (RF) discharge on an I−-CIMS system, providing another alternative to radioactive sources albeit with lower signal levels. However, the RF source also generated high levels of other ions such as dicyanoiodate anion , which can lead to interference but also provide additional pathways for detecting species such as SO2 and HCl.
In this work, we investigate the use of a small krypton (Kr) lamp as a substitute for a radioactive ion source on an I−-CIMS. Similar lamps have been commonly used in atmospheric pressure photoionization–mass spectrometry (e.g., Kauppila et al., 2017). The vacuum ultraviolet (VUV) light is generated from two emission lines centered at 116.486 (photon energy = 10.641 eV) and 123.582 nm (photon energy = 10.030 eV). CH3I has a large absorption cross section ( cm2 molecule−1) at these wavelengths and a relatively low ionization potential ( eV) (Holmes and Lossing, 1991; Olney et al., 1998). Absorption of the VUV light by CH3I forms cations and relatively low-energy photoelectrons, which can then attach to CH3I to form I−. Benzene (C6H6) can also serve as a VUV absorber to produce photoelectrons as it has a larger absorption cross section (4 × 10−17 cm2 molecule−1) and an even lower IP (9.24378±0.00007 eV) (Nemeth et al., 1993; Capalbo et al., 2016). We explore the use of C6H6 as a source of photoelectrons as we have found that delivering even modest quantities of gas-phase CH3I to our ion source can be problematic as it has a tendency to polymerize to nonvolatile species in compressed gas cylinders. Tests of C6H6 as a photoelectron source in this work are performed as it may enhance ion production when used in combination with lower levels of CH3I. C6H6 may also be used as an electron source for use with other electron-attaching compounds with higher IPs, such as SF6, to form reagent ions such as .
The performance of the vacuum ultraviolet ion source (VUV-IS) was characterized by measuring the sensitivities on a quadrupole CIMS (Q-CIMS) to formic acid, chlorine (Cl2), and nitryl chloride (ClNO2), under different flow conditions with varying levels of CH3I. Similar tests were also performed using a flow of both C6H6 and CH3I through the ion source. Potential interferences due to the VUV light interacting with air or surfaces are investigated by comparing ambient mass spectra obtained with a VUV-IS and a standard 20 mCi 210Po ion source (NRD Static Control, P-2031) on both a commercial high-resolution time-of-flight CIMS (TOF-CIMS) and a Q-CIMS. We also test the potential of measuring PAN using this VUV-IS on a TD (thermal dissociation)-CIMS. The reliability of the VUV-IS is tested by performing field measurements for a 6-week time period at a remote location. The potential of extending the use of VUV-IS to and airborne operation is also explored.
2.1 Quadrupole I−-CIMS and experimental configurations
The Q-CIMS used here is very similar to the system that has measured a variety of species, such as Cl2, BrO, and PAN, and has been detailed in previous publications (Slusher et al., 2004; Liao et al., 2011, 2014; Lee et al., 2020). Details specific to these experiments are described below. A diagram of the I−-CIMS system and the experimental layout is shown in Fig. 1. Varying levels of calibration standard were added to 4–10 standard liters per minute (sLpm) of N2 and delivered to the sampling inlet of the CIMS through perfluoroalkoxy (PFA) Teflon tubing, with dimensions of 1.27 cm outer diameter and 0.95 cm inner diameter. Approximately 1.6 sLpm of this flow was sampled into the CIMS flow tube and the rest was exhausted into the lab. The flow tube was humidified by adding 20 standard cubic centimeters per minute (sccm) of N2 through a water bubbler kept in an ice bath. The flow tube was operated at a pressure of either 27 or 53 hPa by using either a 0.91 or 0.635 mm orifice between the flow tube and the collisional dissociation chamber (CDC). The scroll pump flow was controlled to maintain the flow tube at 27 or 53 hPa.
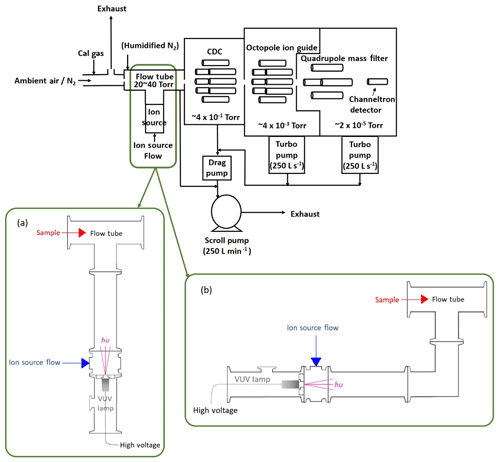
Figure 1Diagram of the I−-Q-CIMS system with a VUV-IS in two different configurations. Configuration (a) provides the most direct route for the generated ions but also directly illuminates the flow tube. Configuration (b) shields the flow tube from the VUV photons by inserting a QF 40 elbow between the photoionization region and the flow tube.
Mass spectra of ambient Atlanta air were obtained in order to check for potential interferences due to the VUV-IS. For these experiments air was sampled from the roof of the Environmental Science and Technology building on the Georgia Tech campus. A PFA Teflon tube of 0.95 cm inner diameter and 8 m length was used as a sampling inlet. A total flow of ∼7 sLpm of ambient air was drawn through the inlet, of which 1.7 sLpm was sampled into the CIMS flow tube and the rest was exhausted through a diaphragm pump. The flow tube was controlled at 27 hPa.
2.2 Calibration sources
Permeation tubes (KIN-TEK Laboratories, Inc.) were used as the sources of Cl2 and formic acid for tests on the Q-CIMS. The output of the formic acid tube was measured by ion chromatography (Metrohm Herisau, 761 Compact IC) as detailed by Nah et al. (2018). The Cl2 permeation tube emission rate was measured by conversion to in aqueous solution, and the resulting was quantified by optical absorption at 352 nm on a spectrophotometer (Finley and Saltzman, 2008). The permeation rates were measured to be 104.7±7.8 ng min−1 for formic acid and 14.8±1.2 ng min−1 for Cl2. A ClNO2 standard was generated by passing a humidified flow of Cl2 from the permeation tube in N2 through a bed of sodium nitrite (NaNO2). The yield of ClNO2 from Cl2 was assumed to be 50 % as we have consistently found in previous studies (Liu et al., 2017).
Sensitivity tests to formic acid and Cl2 on a TOF-CIMS were performed by standard addition in laboratory air. The calibration species were obtained from a calibrated formic acid permeation device (47 ng min−1) and a 4 ppm Cl2 in N2 compressed gas cylinder. The Cl2 cylinder was calibrated by cavity ring-down spectroscopy (CRDS) at 405 nm. The permeation rate of formic acid was measured by catalytic conversion to CO2 followed by CO2 detection as detailed by Veres et al. (2010).
2.3 VUV ion source (VUV-IS)
In a typical I−-CIMS system, a flow of CH3I in N2 passes through a 210Po radioactive ion source to form the reagent ion I−. In this study, the radioactive source was removed and replaced with a VUV lamp assembly. The Kr lamp is powered by a 4 W DC power supply (UltraVolt® AA Series High-Voltage Biasing Supplies). Two configurations (a and b, as shown in Fig. 1) of the VUV lamp assembly were tested. In both configurations a small Kr VUV lamp (Heraeus, Type No. PKS 106) (19.6 mm diameter × 53.5 mm length) was used to generate ions. This lamp is commonly used in small commercial volatile organic compound (VOC) detectors that utilize photoionization as a detection method. The lifetime of this lamp is estimated to be 4000 h (∼5.5 months of continuous use) by the manufacturer. The VUV lamp was operated at ∼280 V DC and typically drew ∼0.7 mA. In general, the ion current reaching the mass spectrometer increased with increasing lamp voltage (see Fig. S1 in the Supplement). The VUV lamp was attached to a custom QF 40 centering ring with vacuum epoxy. The centering ring has a through hole (11.4 mm diameter) with a counterbore (41.1 mm diameter) in the center. The lamp is sealed to the edge of the counterbore with vacuum epoxy. The through hole allows light from the lamp to enter the ion source region of the CIMS. The QF 40 centering ring is mated on the low-pressure side to an inline tee with two QF 40 flanges on the ends and a 0.635 cm National Pipe Thread (NPT) fitting in the center. In lamp configuration (a), the inline tee is attached to a standard short QF 40 nipple (126 mm length) which serves as a photoionization region and provides a direct path for the VUV photons and generated ions into the flow tube. In configuration (b), a QF 40 90∘ elbow was attached between the QF 40 nipple and CIMS flow tube to prevent direct exposure of the flow tube to the VUV photons. The ambient pressure side of the QF 40 centering ring, on which the VUV lamp attached, is mated to a QF 40 × QF 16 × QF 40, reducing tee housing for protection of the VUV lamp. The QF 16 branch of the tee enables visual inspection of the lamp operation.
Table 1Experiment conditions, sensitivities, and limits of detection (LODs) for VUV-IS configuration (b).
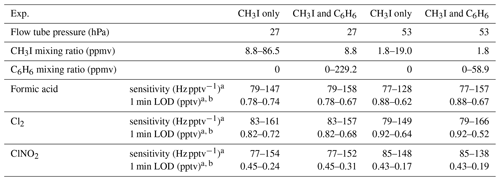
a Sensitivities and detection limits are for HCOOH ⋅ I− ( amu), Cl2 ⋅ I− ( amu), and ClNO2 ⋅ I− ( amu).
b Signal to noise ratio .
Table 2Experiment conditions, sensitivities and limits of detection (LODs) for VUV-IS configuration (a).
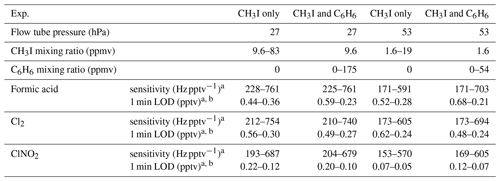
a Sensitivities and detection limits are for HCOOH ⋅ I− (m∕z = 173 amu), Cl2 ⋅ I− ( amu), and ClNO2 ⋅ I− ( amu).
b Signal to noise ratio = 3:1.
The sensitivity of the VUV-IS for measuring formic acid, Cl2, and ClNO2 was measured for varying CH3I concentrations and at two flow tube pressures (Tables 1 and 2). The impact of adding C6H6 was investigated by varying the concentration of C6H6 at a lower level of CH3I. Compressed gas cylinders of ∼700 ppmv of CH3I and ∼0.1 % of C6H6 in N2 were used as CH3I and C6H6 sources. A variable flow of N2 containing CH3I was delivered to the ion source to determine the optimal flow (Fig. 2). The total ion source flow was regulated at 1 sLpm for lamp configuration (a) and 1.2 sLpm for lamp configuration (b) in all sensitivity and interference tests. Mixing ratios of CH3I and C6H6 mentioned in the following sections are the mixing ratios in the total ion source flow (1 or 1.2 sLpm).
2.4 TOF-CIMS
The VUV-IS was also characterized by operation on a commercial TOF-CIMS (Aerodyne Research Incorporated) (Lee et al., 2014; Veres et al., 2020). The ion molecule reactor (IMR) used here was constructed from a 150 mm long QF 40 adapter tee with a 9.5 mm fitting in the center to allow mounting of the VUV-IS. The VUV lamp mounted to the QF 40 inline tee (Sect. 2.1) was attached to a QF 40 × QF 16 conical adapter connected to a flange with a 9.5 mm stainless steel tube. This allowed the VUV-IS assembly to be mated directly to the IMR using standard vacuum components. The IMR was maintained at a pressure of 40 hPa and operated at a total flow of 2.2 sLpm. A 1 sLpm N2 flow with 30–400 ppmv CH3I passed through the VUV-IS into the IMR and mixed with 1.2 sLpm of ambient air. Water was dynamically added to the IMR to maintain a constant ratio of I− to I−(H2O). This provided real-time compensation for changes in ambient humidity to minimize fluctuations in sensitivity. Mass spectra were obtained with both a VUV-IS and a standard radioactive ion source, sampling ambient air in Boulder, Colorado.
2.5 TD-CIMS
The sensitivity of TD-CIMS with a VUV-IS (Slusher et al., 2004) was also tested for PAN. The configuration of the TD-CIMS system used in this work is almost identical to that described in Lee et al. (2020) with the radioactive source replaced with the VUV-IS in configuration (b). A known amount of PAN was generated using a photolytic source similar to that described by Warneck and Zerbach (1992). A calibration standard of 1 ppbv of PAN was produced by adding the output of the photolytic source to PAN-free ambient air. PAN-free air was generated by passing ambient air through a QF 40 nipple filled with stainless steel wool heated to 150 ∘C (Flocke et al., 2005).
2.6 -CIMS
The instrument used to test the VUV-IS with as a reagent ion is nearly identical to that used previously to measure BrO on the National Center for Atmospheric Research (NCAR) Gulfstream-V (GV) research aircraft (Chen et al., 2016). The operating parameters of the instrument are very similar to those used previously to simultaneously detect sulfur dioxide, formic acid, and acetic acid (Nah et al., 2018). However, in this application the radioactive ion source was replaced with a VUV-IS in configuration (b). The system was periodically calibrated in-flight by adding a known amount of isotopically labeled 34SO2 into the sampled air flow.
3.1 Q-CIMS sensitivities using CH3I
The sensitivities and LODs for formic acid, Cl2, and ClNO2 under different experimental conditions using lamp configuration (b) are compiled in Table 1. With the flow tube at 27 hPa, sensitivities to formic acid, Cl2, and ClNO2 reached up to 147, 161, and 154 Hz pptv−1, respectively, using up to 86.5 ppmv of CH3I in the ion source flow. At 53 hPa, similar sensitivities (128, 149, and 148 Hz pptv−1 for formic acid, Cl2, and ClNO2, respectively) were achieved with less CH3I (19.0 ppmv). Figure 3 shows the dependence of the CIMS sensitivities on the CH3I level at 27 hPa (i) and 53 hPa (iii) with no other absorber added. In general, CIMS sensitivities increase with the CH3I mixing ratio. However, the response is less than linear and appears to saturate at higher levels of absorber. With the maximum concentration of CH3I (86.5 ppmv, 5.70 × 1013 molecule cm−3) used in this experiment, ∼8 % of photons emitted from the VUV lamp were absorbed (see the Supplement for sample calculation). This indicates that other factors such as ion recombination and wall loss limit the ion abundance. The sensitivities and LODs under similar experimental conditions using lamp configuration (a) are shown in Table 2 and Fig. 4. The sensitivities to formic acid, Cl2, and ClNO2 approached ∼700 Hz pptv−1, with limits of detection of less than 1 pptv for a 1 min integration period. Limits of detection are defined at a signal-to-noise ratio of 3 where the noise is the variance of the background measurements. In general, the sensitivities using lamp configuration (a) were about a factor of 4 larger than in configuration (b). Note that all the Q-CIMS sensitivities reported in this work are not normalized to the reagent ion signal, since the reagent ion signal is not known accurately. We estimate the reagent ion signals are ∼100 MHz at the highest sensitivities, but the Q-CIMS detector counts ions linearly only up to about 0.5 MHz.
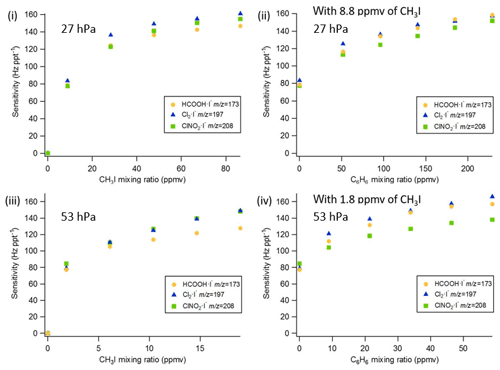
Figure 3Q-CIMS using VUV-IS configuration (b): (i) sensitivity as a function of CH3I at 27 hPa, (ii) sensitivity as a function of C6H6 at 27 hPa with 8.8 ppmv of CH3I, (iii) sensitivity as a function of CH3I at 53 hPa, and (iv) sensitivity as a function of C6H6 at 53 hPa with 1.8 ppmv of CH3I.
3.2 Q-CIMS sensitivities using CH3I and C6H6
In order to test the effectiveness of the addition of another absorber to generate photoelectrons, mixtures of CH3I and C6H6 were added to the ion source. Lower mixing ratios (8.8 ppmv at 20 Torr, 1.8 ppmv at 40 Torr) of CH3I were used in combination with varying amounts of C6H6 to assess the impact of the addition of C6H6 to the generated ion current using lamp configuration (b). The sensitivity dependence on C6H6 mixing ratio is shown in Fig. 3. At 27 hPa, up to 229.2 ppmv C6H6 was added to 8.8 ppmv CH3I to achieve the equivalent sensitivity (158, 157, and 152 Hz pptv−1 for formic acid, Cl2 and ClNO2, respectively) using 86.5 ppmv CH3I alone. At 53 hPa, up to 58.9 ppmv C6H6 was added to 1.8 ppmv of CH3I to reach the maximum level of sensitivities (157, 166, and 138 Hz pptv−1 for formic acid, Cl2 and ClNO2, respectively) when using 19.0 ppmv of CH3I. Similar trends for the addition of C6H6 using lamp configuration (a) was observed and are shown in Table 2 and Fig. 4. At both 27 and 53 hPa sensitivities of more than 600 Hz pptv−1 were obtained for all species in configuration (a). These results demonstrate that addition of an absorber (e.g., C6H6) enables high sensitivity with the VUV-IS while adding typical levels (a few ppmv) of an electron-attaching compound.
3.3 Q-CIMS interference tests
Representative mass spectra ( 20–220 amu) taken with a 20 mCi 210Po standard radioactive source and the VUV-IS with configuration (a) on an I−-CIMS sampling ambient air are shown in Fig. 5. Note that the I− signal is saturated in all mass spectra due to the very high signal levels. Clearly, the VUV-IS in configuration (a) generates many additional ions compared to a radioactive source. Large signals (>100 000 Hz) are observed at ( amu), ( amu), and ( amu). This indicates that photoelectrons generated on the illuminated surface of the flow tube in the presence of the sampled air leads to formation of by electron attachment to O2. This also leads to the formation of and by subsequent reactions with CO2, O3, and NO2 (Mohler and Arnold, 1991). Consequently, the generation of initiates significant secondary chemistry that may lead to interfering signals at a large number of masses.
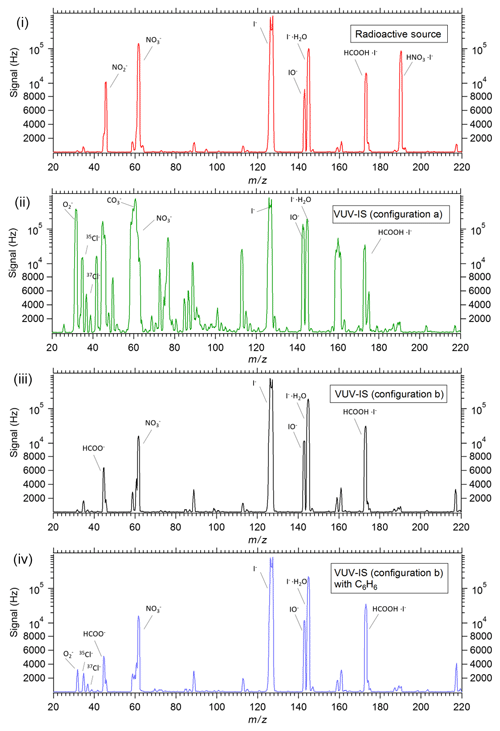
Figure 5Mass spectra of ambient air from a Q-CIMS with (i) a standard radioactive ion source, (ii) VUV-IS in configuration (a), (iii) VUV-IS in configuration (b), and (iv) VUV-IS in configuration (b) with ∼100 ppmv of C6H6 and ∼10 ppmv of CH3I. Note that the I− signal is saturated in all mass spectra.
Using the VUV-IS in configuration (b) prevents direct illumination of the flow tube by the VUV lamp and produces similar spectra to those obtained with a radioactive source (Fig. 5). The signal levels are lower by more than 3 orders of magnitude compared to configuration (a) where the flow tube is directly illuminated.
Finally, Fig. 5 also has a mass spectrum using the VUV-IS in configuration (b) with the addition of 110 ppmv of C6H6 and 8.8 ppmv of CH3I in the ion source flow. The addition of the C6H6 does not produce significant amounts of new ions, and the mass spectrum is very similar to that obtained without C6H6. This indicates that using C6H6 (or other low IP compounds such as toluene or propene) as a light absorber to generate photoelectrons has the potential to extend the use of the VUV-IS to other electron-attaching compounds (e.g., SF6, HNO3, etc.) that have small absorption cross sections in the VUV or have ionization potentials higher than 10.6 eV.
3.4 Q-CIMS field tests
Ground-based measurements of ClNO2, dinitrogen pentoxide (N2O5) and formic acid using the I−-CIMS with the VUV-IS were conducted at a rural site in Dongying, China, during the Ozone Photochemistry and Export from China Experiment (OPECE) from 20 March to 22 April 2018. The I−-CIMS was deployed in a shelter, with neither heating nor air conditioning, in a remote location in a bird sanctuary in the Yellow River Delta. The site experienced intermittent power interruptions and large ambient temperature variations, from −2.5 to 29.1 ∘C, with the temperature inside the shelter ranging from ∼10 to 40 ∘C.
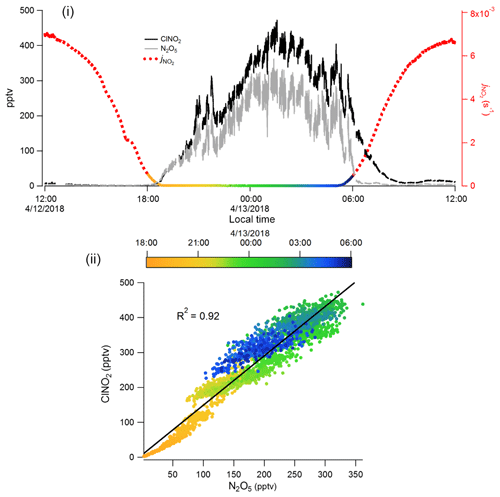
Figure 6Measurements of ClNO2 and N2O5 using I−-CIMS with VUV-IS between 12:00 LT, 12 April and 12:00 LT, 13 April 2018 during the OPECE campaign: (i) time series, along with (to delineate night and day), and (ii) a correlation plot of ClNO2 concentration versus N2O5 concentration during the nighttime (18:00 LT, 12 April, to 06:00 LT, 13 April).
The primary goal for the I−-CIMS during the OPECE campaign was to measure halogen-containing compounds. However, we found the presence of halogens to be intermittent. Figure 6 shows representative observations of ClNO2 and N2O5 for this site when halogens were observed. This figure is consistent with the expected behavior of ClNO2 and N2O5, both accumulate during nighttime and both decrease after sunrise due to photolysis of ClNO2 and thermal decomposition of N2O5, followed by photolysis of NO3. ClNO2 is a product of reaction between N2O5 and chloride-containing aerosol (Finlayson-Pitts et al., 1989), and ClNO2 and N2O5 are well correlated (R2 = 0.94, Fig. 6ii) during the night (18:00 LT, 12 April to 06:00 LT, 13 April). These measurements of ClNO2 and N2O5 indicate the performance of the I−-CIMS with the VUV-IS is sufficient to capture atmospheric levels and variability.
Formic acid, which is routinely measured by I−-CIMS and ubiquitous in the atmosphere as both an emission and a secondary chemical product, was also monitored during the campaign. These observations demonstrate that I−-CIMS with a VUV-IS could be operated continuously for an extended period (Fig. 7). A clogged mass flow controller on the inlet and a scroll pump failure caused brief measurement interruptions on 27 March and in early April. We could not obtain gas mixtures of CH3I at this field location, so we used a liquid reservoir as a CH3I source. This led to using CH3I levels of hundreds of ppmv, which may have accelerated degradation of the scroll pump tip seals. We also encountered some temperature control issues and power interruptions during the mission. However, no direct problems were encountered with the VUV-IS. Online calibration of formic acid was performed every 30 min during the mission. The CIMS sensitivity to formic acid was measured to be 185.2±48.3 Hz pptv−1 during the first day and 180.5±24.3 Hz pptv−1 a month later, so we did not notice any drop in sensitivity that could be attributed to a decrease in light intensity from the lamp. In addition, we have used the same VUV-IS since the OPECE field mission (spring 2018) through early 2020 for both lab studies and field measurements and have not found obvious sensitivity degradations.
3.5 TOF-CIMS tests
The VUV-IS was found to give very similar signal levels to those obtained with a standard radioactive ion source (with an activity of ∼16 mCi of 210Po) on a TOF-CIMS. Both sources gave total reagent ion signals of ∼10 MHz and similar sensitivity to both formic acid and Cl2 of about 20–25 Hz ppt−1. The I− signal for both sources was ∼6 MHz, and the I−(H2O) signal was ∼3 MHz. In addition, as illustrated in Fig. 8, both sources gave very similar mass spectra for ambient air. The largest difference between the mass spectra was that the VUV-IS gave higher levels of , while the radioactive source gave higher levels of peaks corresponding to nitric acid (i.e., I−(HNO3) and ). Sensitivities as a function of CH3I mixing ratio in the flow tube were also tested (Fig. S2). Sensitivities to formic acid and Cl2 increase with the CH3I mixing ratio up to ∼100 ppmv. The sensitivities normalized to the reagent ion I−(H2O) do not change with CH3I mixing ratio and are the same as those obtained with the radioactive source. These results demonstrate that a VUV-IS can be used on a TOF-CIMS to obtain the same sensitivity, selectivity, and ion distribution as with a radioactive ion source.
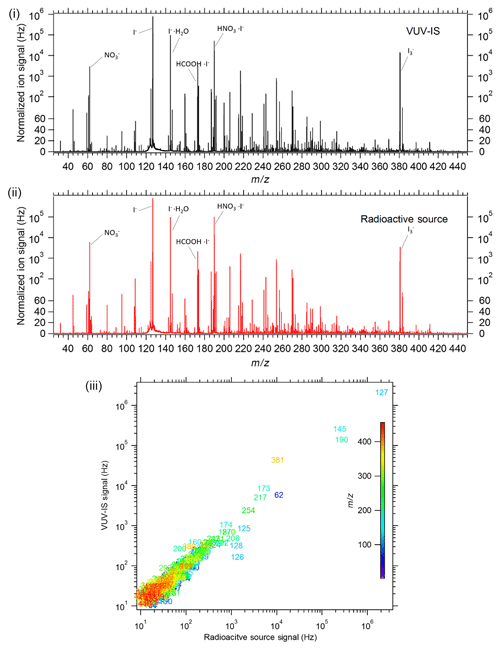
Figure 8Mass spectra of ambient air in Boulder, Colorado, from a TOF-CIMS with (i) a VUV-IS and (ii) a radioactive source. The bottom panel (iii) is a correlation plot of the individual mass signals with the VUV-IS versus those obtained with a radioactive source, with each ion labeled by its nominal mass.
3.6 PAN measurement tests
Preliminary tests using the Q-CIMS as a TD-CIMS demonstrated the potential of the VUV-IS for use in the measurement of PAN. Mass spectra of ambient air with and without PAN are shown in Fig. S3. The sensitivity towards PAN was observed to be 49.4 Hz pptv−1 with an LOD (signal-to-noise ratio ) of 0.64 pptv for a 1 min integration. No significant interferences were observed during the ambient air tests.
3.7 -Q-CIMS tests
Preliminary aircraft-based measurements of sulfur dioxide and formic and acetic acid using the -CIMS with the VUV-IS were conducted during an Asian Summer Monsoon Chemical and Climate Impact Project (ACCLIP) test flight based out of Broomfield, Colorado, on 30 January 2020. Time series of formic and acetic acid signals are shown in Fig. S4(1). The signals of formic and acetic acid are correlated (R2 = 0.63, Fig. S4(2)) as observed in previous studies (Souza and Carvalho, 2001; Paulot et al., 2011; Nah et al., 2018). We did not perform online calibrations for formic or acetic acid. Sensitivities to formic and acetic acids during this test flight are estimated to be 5–20 Hz pptv−1 based on online calibrations of 34SO2 and measurements of the ratio of their sensitivities (Nah et al., 2018).
The VUV-IS can generate I− ions and mass spectra that are very similar to a radioactive ion source on both a TOF-CIMS and a Q-CIMS. For this reason, we think that the VUV-IS can replace radioactive ion sources in most I−-CIMS applications without any loss of measurement performance. Perhaps the largest benefit of the VUV-IS is that it will expand the use of I−-CIMS to locations or situations where radioactivity is not allowed. The VUV-IS is also likely to be useful for laboratory flow tube or chamber studies that use CIMS as a detector (D'Ambro et al., 2017; Huang et al., 2017; Faxon et al., 2018). We have not found any interferences or issues that would limit application of a VUV-IS, though its application to the measurement of individual species must be confirmed by further testing.
Using the VUV-IS requires attention to the possibility of interferences caused by the generation of photoelectrons from surfaces that can be attached by oxygen or other compounds in the sampled gas matrix. We were able to minimize this effect by using a flow geometry that shielded the flow tube from the VUV photons, albeit at the expense of more than a factor of 4 in signal on the Q-CIMS. Similarly, the implementation of the VUV-IS on the TOF-CIMS successfully limited production by passing the ion source flow through a small diameter tube that discriminated against light reaching the IMR. This issue might also be addressed by further improvements in geometry, the use of optical focusing elements, or higher levels of an absorber molecule.
The VUV-IS source requires an absorbing species to serve as a source of photoelectrons. Extending the use of the VUV-IS to other reagent ions such as (Nah et al., 2018), Br− (Sanchez et al., 2016), (Eisele and Tanner, 1993), CF3O− (Crounse et al., 2006), and (Veres et al., 2008) requires the addition of an absorber such as C6H6 or C7H8. Although the use of CH3I to produce I− with the VUV-IS does not require an additional absorber, this application requires relatively high levels of CH3I to obtain maximum sensitivities. Since CH3I is a hazardous gas, higher levels of CH3I could be problematic for some situations such as deployment in a highly regulated environment such as an aircraft. Higher levels of CH3I may also necessitate protection of scroll pumps with scrubbers and traps. For these reasons, it may be preferable to use a low activity (Lee et al., 2020) or standard radioactive ion source for some applications.
One potential advantage of the VUV-IS is demonstrated by the lower background signals at masses corresponding to nitric acid (HNO3). This is probably due to lower rates of nitrogen radical generation in the VUV-IS as the generated photons are much lower in energy (∼10 eV) relative to the α particles (∼5.4 MeV) from a 210Po radioactive source. Additionally, the VUV-IS does not produce ions when power is removed but radioactive decay is continuous. Consequently, interfering species can be continuously generated in a radioactive source, leading to a build up of condensable species such as nitric acid. For these reasons, the VUV-IS may generate significantly lower levels of interferences than a radioactive source.
The sensitivity of the VUV-IS on the Q-CIMS can reach 100 s of Hz pptv−1 (Tables 1 and 2) similar to the best sensitivity obtained with radioactive sources (e.g., Lee et al., 2020). Care must be taken to avoid illuminating surfaces in the CIMS exposed to air. The VUV-IS requires significantly higher levels of CH3I than used in radioactive sources (typically ∼1 ppmv, Slusher et al., 2004) (Figs. 3 and 4) because the CH3I must also serve as a source of photoelectrons. C6H6 and other species can be used as a VUV absorber to generate photoelectrons (Figs. 3 and 4) without generating excessive interferences (Fig. 5). Tests on a TOF-CIMS demonstrated that the VUV-IS and a standard radioactive ion source produced the same reagent ion (I−) abundance. In addition, both sources generated similar mass spectra of ambient air, demonstrating that the VUV-IS source did not produce significant interferences to the detection of most trace gases (Fig. 8). Preliminary tests also indicate the VUV-IS is compatible with TD-CIMS and -CIMS methods, as well as airborne operation.
The VUV-IS described in this work provides sensitivities and limits of detections that are at least comparable to those obtained with a radioactive ion source for both Q-CIMS and TOF-CIMS using I− as a reagent ion. The VUV-IS is reliable and can be safely deployed in remote field missions. These results demonstrate that the VUV-IS can eliminate the need for radioactivity with an I−-CIMS for most applications. The use of low IP absorbers, such as C6H6, to generate photoelectrons in conjunction with high IP electron-attaching compounds allows the generation of other reagent ions such as , CF3O−, and . In summary, the VUV-IS has the potential to eliminate most of the use of radioactivity with CIMS instruments.
All of the data used in this work are available upon request of the corresponding author.
The supplement related to this article is available online at: https://doi.org/10.5194/amt-13-3683-2020-supplement.
YJ performed all of the Q-CIMS experiments and wrote the manuscript with assistance from LGH. DJT assisted with all of the experiments. XW organized the OPECE campaign and provided the data. All of the authors were involved in data interpretation and commented on the manuscript. The TOF-CIMS experiments were performed by PRV, JAN, and LGH.
The authors declare that they have no conflict of interest.
This work was supported by the NSF and NASA. The OPECE field mission was supported by the NSF. This work was also supported in part by an EPA STAR grant awarded to the Georgia Institute of Technology. It has not been formally reviewed by the EPA. The views expressed in this document are solely those of the authors and do not necessarily reflect those of the EPA. The EPA does not endorse any products or commercial services mentioned in this publication and EPA grant. We thank Jianhui Tang for providing the RH data on the field. We also thank the Yellow River Delta Ecological Research Station of Coastal Wetland, which belongs to the Yantai Institute of Coastal Zone Research, Chinese Academy of Sciences, for logistical support for the OPECE campaign.
This research has been supported by the National Science Foundation (grant nos. 1853930 and 1743401), the U.S. Environmental Protection Agency (STAR (grant no. R835882)), and the National Aeronautics and Space Administration (grant no. NNX15AT90G).
This paper was edited by Fred Stroh and reviewed by Joachim Franzke and one anonymous referee.
Bertram, T. H., Kimmel, J. R., Crisp, T. A., Ryder, O. S., Yatavelli, R. L. N., Thornton, J. A., Cubison, M. J., Gonin, M., and Worsnop, D. R.: A field-deployable, chemical ionization time-of-flight mass spectrometer, Atmos. Meas. Tech., 4, 1471–1479, https://doi.org/10.5194/amt-4-1471-2011, 2011.
Capalbo, F. J., Benilan, Y., Fray, N., Schwell, M., Champion, N., Es-Sebbar, E. T., Koskinen, T. T., Lehocki, I., and Yelle, R. V.: New benzene absorption cross sections in the VUV, relevance for Titan's upper atmosphere, Icarus, 265, 95–109, https://doi.org/10.1016/j.icarus.2015.10.006, 2016.
Chen, D. X., Huey, L. G., Tanner, D. J., Salawitch, R. J., Anderson, D. C., Wales, P. A., Pan, L. L., Atlas, E. L., Hornbrook, R. S., Apel, E. C., Blake, N. J., Campos, T. L., Donets, V., Flocke, F. M., Hall, S. R., Hanisco, T. F., Hills, A. J., Honomichl, S. B., Jensen, J. B., Kaser, L., Montzka, D. D., Nicely, J. M., Reeves, J. M., Riemer, D. D., Schauffler, S. M., Ullmann, K., Weinheimer, A. J., and Wolfe, G. M.: Airborne measurements of BrO and the sum of HOBr and Br2 over the Tropical West Pacific from 1 to 15 km during the CONvective TRansport of Active Species in the Tropics (CONTRAST) experiment, J. Geophys. Res.-Atmos., 121, 12560–12578, 2016.
Crounse, J. D., McKinney, K. A., Kwan, A. J., and Wennberg, P. O.: Measurement of gas-phase hydroperoxides by chemical ionization mass spectrometry, Anal. Chem., 78, 6726–6732, https://doi.org/10.1021/ac0604235, 2006.
D'Ambro, E. L., Lee, B. H., Liu, J., Shilling, J. E., Gaston, C. J., Lopez-Hilfiker, F. D., Schobesberger, S., Zaveri, R. A., Mohr, C., Lutz, A., Zhang, Z., Gold, A., Surratt, J. D., Rivera-Rios, J. C., Keutsch, F. N., and Thornton, J. A.: Molecular composition and volatility of isoprene photochemical oxidation secondary organic aerosol under low- and high-NOx conditions, Atmos. Chem. Phys., 17, 159–174, https://doi.org/10.5194/acp-17-159-2017, 2017.
Eger, P. G., Helleis, F., Schuster, G., Phillips, G. J., Lelieveld, J., and Crowley, J. N.: Chemical ionization quadrupole mass spectrometer with an electrical discharge ion source for atmospheric trace gas measurement, Atmos. Meas. Tech., 12, 1935–1954, https://doi.org/10.5194/amt-12-1935-2019, 2019.
Eisele, F. L. and Tanner, D. J.: Measurement of the Gas-Phase Concentration of H2SO4 and Methane Sulfonic-Acid and Estimates of H2SO4 Production and Loss in the Atmosphere, J. Geophys. Res.-Atmos., 98, 9001–9010, https://doi.org/10.1029/93jd00031, 1993.
Faxon, C., Hammes, J., Le Breton, M., Pathak, R. K., and Hallquist, M.: Characterization of organic nitrate constituents of secondary organic aerosol (SOA) from nitrate-radical-initiated oxidation of limonene using high-resolution chemical ionization mass spectrometry, Atmos. Chem. Phys., 18, 5467–5481, https://doi.org/10.5194/acp-18-5467-2018, 2018.
Finlayson-Pitts, B. J., Ezell, M. J. and Pitts, J. N.: Formation of chemically active chlorine compounds by reactions of atmospheric NaCl particles with gaseous N2O5 and ClONO2, Nature, 337, 241–244, 1989.
Finley, B. D. and Saltzman, E. S.: Observations of Cl2, Br2, and I2 in coastal marine air, J. Geophys. Res.-Atmos., 113, D21301, https://doi.org/10.1029/2008JD010269, 2008.
Flocke, F. M., Weinheimer, A. J., Swanson, A. L., Roberts, J. M., Schmitt, R., and Shertz, S.: On the measurement of PANs by gas chromatography and electron capture detection, J. Atmos. Chem., 52, 19–43, https://doi.org/10.1007/s10874-005-6772-0, 2005.
Holmes, J. L. and Lossing, F. P.: Ionization Energies of Homologous Organic-Compounds and Correlation with Molecular-Size, Org. Mass Spectrom., 26, 537–541, DOI https://doi.org/10.1002/oms.1210260603, 1991.
Huang, Y., Coggon, M. M., Zhao, R., Lignell, H., Bauer, M. U., Flagan, R. C., and Seinfeld, J. H.: The Caltech Photooxidation Flow Tube reactor: design, fluid dynamics and characterization, Atmos. Meas. Tech., 10, 839–867, https://doi.org/10.5194/amt-10-839-2017, 2017.
Huey, L. G.: Measurement of trace atmospheric species by chemical ionization mass spectrometry: Speciation of reactive nitrogen and future directions, Mass Spectrometry Reviews, 26, 166–184, https://doi.org/10.1002/mas.20118, 2007.
Jost, C., Sprung, D., Kenntner, T., and Reiner, T.: Atmospheric pressure chemical ionization mass spectrometry for the detection of tropospheric trace gases: the influence of clustering on sensitivity and precision, International Journal of Mass Spectrometry, 223, 771–782, Pii S1387-3806(02)00963-6, Doi https://doi.org/10.1016/S1387-3806(02)00963-6, 2003.
Kauppila, T. J., Syage, J. A., and Benter, T.: Recent Developments in Atmospheric Pressure Photoionization-Mass Spectrometry, Mass Spectrom. Rev., 36, 423–449, 2017.
Kürten, A., Rondo, L., Ehrhart, S., and Curtius, J.: Performance of a corona ion source for measurement of sulfuric acid by chemical ionization mass spectrometry, Atmos. Meas. Tech., 4, 437–443, https://doi.org/10.5194/amt-4-437-2011, 2011.
Lee, B. H., Lopez-Hilfiker, F. D., Mohr, C., Kurten, T., Worsnop, D. R., and Thornton, J. A.: An Iodide-Adduct High-Resolution Time-of-Flight Chemical-Ionization Mass Spectrometer: Application to Atmospheric Inorganic and Organic Compounds, Environ. Sci. Technol., 48, 6309–6317, https://doi.org/10.1021/es500362a, 2014.
Lee, Y. R., Ji, Y., Tanner, D. J., and Huey, L. G.: A low-activity ion source for measurement of atmospheric gases by chemical ionization mass spectrometry, Atmos. Meas. Tech., 13, 2473–2480, https://doi.org/10.5194/amt-13-2473-2020, 2020.
Liao, J., Sihler, H., Huey, L. G., Neuman, J. A., Tanner, D. J., Friess, U., Platt, U., Flocke, F. M., Orlando, J. J., Shepson, P. B., Beine, H. J., Weinheimer, A. J., Sjostedt, S. J., Nowak, J. B., Knapp, D. J., Staebler, R. M., Zheng, W., Sander, R., Hall, S. R., and Ullmann, K.: A comparison of Arctic BrO measurements by chemical ionization mass spectrometry and long path-differential optical absorption spectroscopy, J. Geophys. Res.-Atmos., 116, D00R02, https://doi.org/10.1029/2010jd014788, 2011.
Liao, J., Huey, L. G., Liu, Z., Tanner, D. J., Cantrell, C. A., Orlando, J. J., Flocke, F. M., Shepson, P. B., Weinheimer, A. J., Hall, S. R., Ullmann, K., Beine, H. J., Wang, Y. H., Ingall, E. D., Stephens, C. R., Hornbrook, R. S., Apel, E. C., Riemer, D., Fried, A., Mauldin, R. L., Smith, J. N., Staebler, R. M., Neuman, J. A., and Nowak, J. B.: High levels of molecular chlorine in the Arctic atmosphere, Nat. Geosci., 7, 91–94, https://doi.org/10.1038/Ngeo2046, 2014.
Liu, X., Qu, H., Huey, L. G., Wang, Y., Sjostedt, S., Zeng, L., Lu, K., Wu, Y., Hu, M., Shao, M., Zhu, T., and Zhang, Y.: High Levels of Daytime Molecular Chlorine and Nitryl Chloride at a Rural Site on the North China Plain, Environ. Sci. Technol., 51, 9588–9595, https://doi.org/10.1021/acs.est.7b03039, 2017.
Mohler, O. and Arnold, F.: Flow Reactor and Triple Quadrupole Mass-Spectrometer Investigations of Negative-Ion Reactions Involving Nitric-Acid – Implications for Atmospheric Hno3 Detection by Chemical Ionization Mass-Spectrometry, J. Atmos. Chem., 13, 33–61, https://doi.org/10.1007/Bf00048099, 1991.
Nah, T., Ji, Y., Tanner, D. J., Guo, H., Sullivan, A. P., Ng, N. L., Weber, R. J., and Huey, L. G.: Real-time measurements of gas-phase organic acids using chemical ionization mass spectrometry, Atmos. Meas. Tech., 11, 5087–5104, https://doi.org/10.5194/amt-11-5087-2018, 2018.
Nemeth, G. I., Selzle, H. L., and Schlag, E. W.: Magnetic Zeke Experiments with Mass Analysis, Chem. Phys. Lett., 215, 151–155, https://doi.org/10.1016/0009-2614(93)89279-Q, 1993.
Neuman, J. A., Trainer, M., Brown, S. S., Min, K. E., Nowak, J. B., Parrish, D. D., Peischl, J., Pollack, I. B., Roberts, J. M., Ryerson, T. B., and Veres, P. R.: HONO emission and production determined from airborne measurements over the Southeast US, J. Geophys. Res.-Atmos., 121, 9237–9250, https://doi.org/10.1002/2016jd025197, 2016.
Olney, T. N., Cooper, G., and Brion, C. E.: Quantitative studies of the photoabsorption (4.5–488 eV) and photoionization (9–59.5 eV) of methyl iodide using dipole electron impact techniques, Chem. Phys., 232, 211–237, https://doi.org/10.1016/S0301-0104(97)00368-6, 1998.
Osthoff, H. D., Roberts, J. M., Ravishankara, A. R., Williams, E. J., Lerner, B. M., Sommariva, R., Bates, T. S., Coffman, D., Quinn, P. K., Dibb, J. E., Stark, H., Burkholder, J. B., Talukdar, R. K., Meagher, J., Fehsenfeld, F. C., and Brown, S. S.: High levels of nitryl chloride in the polluted subtropical marine boundary layer, Nat. Geosci., 1, 324–328, https://doi.org/10.1038/ngeo177, 2008.
Phillips, G. J., Pouvesle, N., Thieser, J., Schuster, G., Axinte, R., Fischer, H., Williams, J., Lelieveld, J., and Crowley, J. N.: Peroxyacetyl nitrate (PAN) and peroxyacetic acid (PAA) measurements by iodide chemical ionisation mass spectrometry: first analysis of results in the boreal forest and implications for the measurement of PAN fluxes, Atmos. Chem. Phys., 13, 1129–1139, https://doi.org/10.5194/acp-13-1129-2013, 2013.
Paulot, F., Wunch, D., Crounse, J. D., Toon, G. C., Millet, D. B., DeCarlo, P. F., Vigouroux, C., Deutscher, N. M., González Abad, G., Notholt, J., Warneke, T., Hannigan, J. W., Warneke, C., de Gouw, J. A., Dunlea, E. J., De Mazière, M., Griffith, D. W. T., Bernath, P., Jimenez, J. L., and Wennberg, P. O.: Importance of secondary sources in the atmospheric budgets of formic and acetic acids, Atmos. Chem. Phys., 11, 1989–2013, https://doi.org/10.5194/acp-11-1989-2011, 2011.
Priestley, M., le Breton, M., Bannan, T. J., Worrall, S. D., Bacak, A., Smedley, A. R. D., Reyes-Villegas, E., Mehra, A., Allan, J., Webb, A. R., Shallcross, D. E., Coe, H., and Percival, C. J.: Observations of organic and inorganic chlorinated compounds and their contribution to chlorine radical concentrations in an urban environment in northern Europe during the wintertime, Atmos. Chem. Phys., 18, 13481–13493, https://doi.org/10.5194/acp-18-13481-2018, 2018.
Sanchez, J., Tanner, D. J., Chen, D., Huey, L. G., and Ng, N. L.: A new technique for the direct detection of HO2 radicals using bromide chemical ionization mass spectrometry (Br-CIMS): initial characterization, Atmos. Meas. Tech., 9, 3851–3861, https://doi.org/10.5194/amt-9-3851-2016, 2016.
Skalny, J. D., Horvath, G., and Mason, N. L.: Mass spectrometric analysis of small negative ions (e/m < 100) produced by Trichel pulse negative corona discharge fed by ozonised air, J. Optoelectron. Adv. M., 9, 887–893, 2007.
Slusher, D. L., Huey, L. G., Tanner, D. J., Flocke, F. M., and Roberts, J. M.: A thermal dissociation–chemical ionization mass spectrometry(TD-CIMS) technique for the simultaneous measurement of peroxyacyl nitrates and dinitrogen pentoxide, J. Geophys. Res.-Atmos., 109, D19315, https://doi.org/10.1029/2004JD004670, 2004.
Souza, S. R. and Carvalho, L. R. F.: Seasonality influence in the distribution of formic and acetic acids in the urban atmosphere of Sao Paulo City, Brazil, J. Brazil. Chem. Soc., 12, 755–762, 2001.
Thornton, J. A., Kercher, J. P., Riedel, T. P., Wagner, N. L., Cozic, J., Holloway, J. S., Dube, W. P., Wolfe, G. M., Quinn, P. K., Middlebrook, A. M., Alexander, B., and Brown, S. S.: A large atomic chlorine source inferred from mid-continental reactive nitrogen chemistry, Nature, 464, 271–274, https://doi.org/10.1038/nature08905, 2010.
Veres, P., Roberts, J. M., Warneke, C., Welsh-Bon, D., Zahniser, M., Herndon, S., Fall, R., and de Gouw, J.: Development of negative-ion proton-transfer chemical-ionization mass spectrometry (NI-PT-CIMS) for the measurement of gas-phase organic acids in the atmosphere, Int. J. Mass Spectrom., 274, 48–55, https://doi.org/10.1016/j.ijms.2008.04.032, 2008.
Veres, P., Gilman, J. B., Roberts, J. M., Kuster, W. C., Warneke, C., Burling, I. R., and de Gouw, J.: Development and validation of a portable gas phase standard generation and calibration system for volatile organic compounds, Atmos. Meas. Tech., 3, 683–691, https://doi.org/10.5194/amt-3-683-2010, 2010.
Veres, P. R., Neuman, J. A., Bertram, T. H., Assaf, E., Wolfe, G. M., Williamson, C. J., Weinzierl, B., Tilmes, S., Thompson, C. R., Thames, A. B., Schroder, J. C., Saiz-Lopez, A., Rollins, A. W., Roberts, J. M., Price, D., Peischl, J., Nault, B. A., Moller, K. H., Miller, D. O., Meinardi, S., Li, Q. Y., Lamarque, J. F., Kupc, A., Kjaergaard, H. G., Kinnison, D., Jimenez, J. L., Jernigan, C. M., Hornbrook, R. S., Hills, A., Dollner, M., Day, D. A., Cuevas, C. A., Campuzano-Jost, P., Burkholder, J., Bui, T. P., Brune, W. H., Brown, S. S., Brock, C. A., Bourgeois, I., Blake, D. R., Apel, E. C., and Ryerson, T. B.: Global airborne sampling reveals a previously unobserved dimethyl sulfide oxidation mechanism in the marine atmosphere, P. Natl. Acad. Sci. USA, 117, 4505–4510, 2020.
Wang, X. F., Wang, T., Xue, L. K., Nie, W., Xu, Z., Poon, S. C. N., and Wang, W. X.: Peroxyacetyl nitrate measurements by thermal dissociation-chemical ionization mass spectrometry in an urban environment: performance and characterizations, Front. Env. Sci. Eng., 11, 3, https://doi.org/10.1007/s11783-017-0925-7, 2017.
Warneck, P. and Zerbach, T.: Synthesis of peroxyacetyl nitrate in air by acetone photolysis, Environ. Sci. Technol., 26, 74–79, 1992.