the Creative Commons Attribution 4.0 License.
the Creative Commons Attribution 4.0 License.
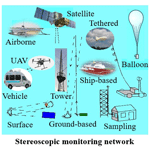
Monitoring greenhouse gases (GHGs) in China: status and perspective
Youwen Sun
Hao Yin
Wei Wang
Changgong Shan
Justus Notholt
Mathias Palm
Ke Liu
In order to establish a creditable greenhouse gas (GHG) monitoring network to support the goals of carbon peak/neutrality, it is necessary to know what we have done and what we have to do in the future. In this study, we summarize an overview of the status and perspective of GHG monitoring in China. With decades of effort, China has made a great breakthrough in GHG monitoring capacity and steadily improved the performance of homemade GHG monitoring instruments. However, most GHG monitoring studies have been research-oriented, temporal, sparse, and uncoordinated. It is suggested to take full advantage of various monitoring technologies, monitoring platforms, numerical simulations, and inventory compilation techniques to form a creditable GHG stereoscopic monitoring and assessment system at an operational level. We envisage that this system can routinely quantify GHGs on national, provincial, regional, and even individual scales with high spatiotemporal resolution and wide coverage to support low-carbon policy in China.
- Article
(3453 KB) - Full-text XML
-
Supplement
(581 KB) - BibTeX
- EndNote
Climate change is one of the great challenges facing humankind around the globe (Tian et al., 2017; Sun et al., 2021; Liu et al., 2022). According to the United Nations Intergovernmental Panel on Climate Change (IPCC) estimates, in order to achieve the 1.5 ∘C target of the Paris Agreement, the integrated Earth system must achieve net-zero carbon dioxide (CO2) emissions (also known as “neutrality”) by 2050; i.e., the annual CO2 emissions are equal to the amount of CO2 reduction through strategies that can either increase carbon sinks or reduce carbon sources, e.g., decarbonization, carbon offset, energy consumption reduction, tree planting, carbon capture and storage (CCS), and carbon sequestration (Zheng et al., 2020a). Carbon neutrality is an important strategy to tackle global climate change (IPCC, 2019). Currently, 137 countries around the globe have proposed carbon neutrality deadlines through policy announcements or legislation. Most of these countries, such as the ones from the European Union, the United States, Japan, Great Britain, Australia, Canada, New Zealand, and South Africa, have committed to achieving carbon neutrality by 2050 (IPCC, 2019). A few countries such as Germany have brought forward their carbon neutrality deadline to 2045 (IPCC, 2019). Since most developed countries have already achieved carbon peak, they only need to continue their previous greenhouse gas (GHG) reduction strategies for achieving their carbon neutrality goals, and thus their carbon reduction tasks are relatively easy to realize (IPCC, 2019). Although the total carbon emissions are still increasing, China is committed to achieving the goals of carbon peak by 2030 and carbon neutrality by 2060 (Liu et al., 2022). Considering much of China's economic growth still relies on high-carbon energy previously implemented by developed countries, the next 30 years will be a critical period for China to balance its economic development with industrial transformation. During the 14th Five-Year Plan stage, China's ecological civilization construction will step into a critical stage for upgrading its ecological environment quality (Zhao et al., 2021). In addition to continuing current pollution control policies, this stage will promulgate a series of carbon reduction measures to achieve an initial low-carbon transformation for economic and social development (Yang et al., 2021a).
To tackle climate change, it is critical to have creditable information on GHGs with respect to who, which emission sector, and what quantity are responsible for the emissions (Boesch et al., 2021). This information allows assessment of the effectiveness of GHG mitigation initiatives, strategies, and policies. In particular it allows assessment of how much GHG reductions are being met at global, national, sector, or even individual point sources. It also allows GHG trading schemes to be functional since such schemes would have no integrity without credible trading units. This credibility determines if buyers and sellers will have confidence in such trading schemes (Boesch et al., 2021). Accurate GHG information is also crucial for investigating the relationship between global warming and GHGs (Wunch et al., 2010, 2011). By accurately capturing the diurnal, monthly, seasonal, and inter-annual variabilities of key GHGs, we can speculate on their sources and sinks, reveal the physical and chemical mechanisms that drive their variabilities, predict their future trends, and understand how GHG emissions interact with the atmosphere and how the climate responds to both natural and anthropogenic GHG emissions (Wunch et al., 2011). In addition, a creditable GHG monitoring system could not only promote the investigation of the carbon cycle, but also support the development of chemical transport models (CTMs) and emission inventory compilation technology around the globe (MacFaul, 2007; Z. D. Yang et al., 2020).
In order to establish a creditable GHG monitoring network to support the goals of carbon peak/neutrality in China, it is necessary to know what we have done and what we have to do in the future. In this study, we summarize an overview of the status and perspective of GHG monitoring in China. There is a very large number of topics and literature related to GHGs, and this work cannot summarize all of them, but it will attempt to condense the major information in the field of GHG monitoring capacity in China. In Sect. 2, we briefly introduce the history of GHG monitoring around the globe. Sections 3 and 4 summarize the status and typical advances of GHG monitoring in China. Section 5 discusses the main challenges that need to be addressed for developing a creditable GHG stereoscopic monitoring network in China. In Sect. 6, we present a perspective for future development of GHG monitoring in China. Section 7 gives the conclusions.
The first continuous monitoring of atmospheric GHGs was started at Mauna Loa (19.5∘ N, 155.6∘ W) in Hawaii in 1957 (MacFaul, 2007). In situ GHG measurements at this station were based on nondispersive infrared (NDIR) spectroscopic technology and were conducted by the National Oceanic and Atmospheric Administration (NOAA) of the United States. Subsequently, NOAA expanded such continuous routine monitoring of atmospheric GHGs to Barrow (71.3∘ N, 156.6∘ W), American Samoa (14.2∘ S, 170.6∘ W), and the South Pole (90.0∘ S, 59.0∘ E) (MacFaul, 2007). Long-term time series of GHG measurements at the four observatories show that global atmospheric CO2 concentration increased year by year in the past 50 years (Fig. 1). Furthermore, the Global Atmosphere Watch (GAW) network organized by the World Meteorological Organization (WMO) measures atmospheric GHGs from several ground-based and tower-based stations around the globe (Fig. 2). Currently, the GAW only operates one global station (Mt. Waliguan (36.3∘ N, 100.9∘ E)) and three regional stations (Lin'an (30.3∘ N, 119.7∘ E), Longfengshan (44.7∘ N, 127.6∘ E), and Shangdianzi (40.7∘ N, 117.2∘ E)) within China (Fang et al., 2014, 2015a, b, 2016). Most GAW ground-based and tower-based stations use commercially available cavity ring-down spectroscopic (CRDS) instruments to achieve high-precision measurements of GHGs (Gomez-Pelaez et al., 2019). Furthermore, GAW also operates many airborne in situ monitoring instrumentations for atmospheric GHG monitoring around the globe (Fig. 2). These airborne measurement campaigns include the Intercontinental Chemical Transport Experiment–North America campaign (INTEX-NA) and the CO2 Budget and Rectification Airborne – Maine experiment (COBRA-ME) over the United States during 2004–2005 (Gerbig et al., 2003; Lin et al., 2006; Singh et al., 2006); the Tropical Warm Pool International Cloud Experiment (TWP-ICE) over Australia in 2006; the HIAPER aircraft campaign following the START-08 and HIPPO campaigns in 2008 and 2009; the Beechcraft King Air aircraft campaign over Tsukuba, Japan, in 2009, and Learjet overflights over Lamont, United States, in 2009 (Wunch et al., 2010).
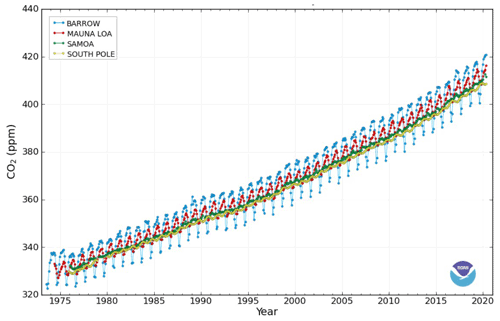
Figure 1Long-term time series of CO2 at the Mauna Loa, Barrow, American Samoa, and South Pole observatories (adapted from https://gml.noaa.gov/, last access: 11 June 2022).
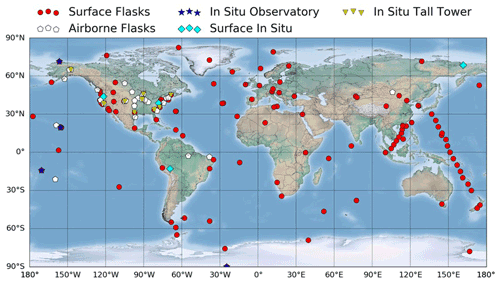
Figure 2Global GHG monitoring network coordinated by NOAA and WMO. Geolocations of all sites are listed in Table S1 in the Supplement. Base map of this figure is from the Basemap package of Python.
Optical remote sensing techniques sampling the total atmospheric column have been developed throughout the last 2 decades and have been found to be very useful for monitoring atmospheric GHGs (Wunch et al., 2011). A series of state-of-the-art satellites with different spatiotemporal resolutions, including SCIAMACHY (Schneising et al., 2012; Dils et al., 2014; Houweling et al., 2014; Buchwitz et al., 2015; Heymann et al., 2015; Kulawik et al., 2016) and TROPOMI (Butz et al., 2012; Veefkind et al., 2012; Pandey et al., 2019, 2021; C. Wang et al., 2020; Y. Z. Zhang et al., 2020; Barre et al., 2021; Park et al., 2021; Qu et al., 2021; Sha et al., 2021; Shen et al., 2021) from the European Space Agency (ESA), GOSAT and GOSAT-2 from Japan (Butz et al., 2011; Morino et al., 2011; Cogan et al., 2012; Yoshida et al., 2013; Deng et al., 2014; Parker et al., 2020; Boesch et al., 2021), OCO-2 and OCO-3 from the United States (Thompson et al., 2012; Frankenberg et al., 2015; Eldering et al., 2017; Nassar et al., 2017; Patra et al., 2017; Wunch et al., 2017; C. Wang et al., 2020; Zheng et al., 2020a, b; Hu and Shi, 2021; Kiel et al., 2021), TanSat (Liu et al., 2013, 2014, 2018; D. X. Yang et al., 2018; Z. D. Yang et al., 2018b; Zhang et al., 2019; Z. D. Yang et al., 2020; Bao et al., 2020; S. P. Wang et al., 2020; D. Yang et al., 2020; Yang et al., 2021a, b) and Gaofen-5 (GF-5) series satellites from China (Li et al., 2016; Wu et al., 2018; X. Y. Zhang et al., 2020; Zhao et al., 2021), GHGSat from Canada (Varon et al., 2019; Jervis et al., 2021), etc., have been launched to derive the global distributions of GHGs. These satellites mainly measure total columns of GHGs by means of infrared grating or Fourier transform infrared (FTIR) spectrometers through atmospheric limb or nadir observations. SCIAMACHY, GOSAT, OCO-2, and TanSat have XCO2 precisions of 2.5, 1–2, ∼1, and 1–4 ppmv, respectively (Reuter et al., 2011; Nassar et al., 2017; Boesch et al., 2021; D. Yang et al., 2020). Studies with satellite data have yielded anthropogenic CO2 flux estimates at the scale of megacities or larger regions (Eldering et al., 2017) and recently have extended CO2 emissions estimates at the scale of an individual facility, such as a single power plant (Nassar et al., 2017; Zheng et al., 2020a). Jacob et al. (2022) have summarized the capability of current and scheduled satellite observations of atmospheric methane in the shortwave infrared (SWIR) to quantify CH4 emissions from the global scale down to point sources, where XCH4 precisions of various satellites were presented.
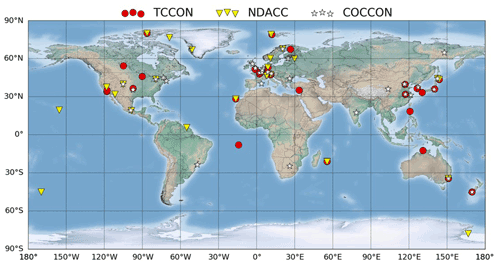
Figure 3Global FTIR observation networks, including TCCON, NDACC-IRWG, and COCCON networks. Geolocations of all sites are listed in Table S2 in the Supplement. Base map of this figure is from the Basemap package of Python.
Ground-based high-resolution FTIR spectrometers are powerful tools for deriving total columns and profiles of GHGs (Wunch et al., 2011). Both the Total Carbon Column Observing Network (TCCON) and the Network for Detection of Atmospheric Composition Change-Infrared working group (NDACC-IRWG) use high-resolution FTIR spectrometers (mainly IFS120HR/IFS125HR series spectrometers manufactured by Bruker, Germany) to observe total columns and profiles of GHGs and atmospheric pollutants (Chevallier et al., 2011; Messerschmidt et al., 2011; Saito et al., 2012; Kuai et al., 2012; Connor et al., 2016; Kiel et al., 2016; Belikov et al., 2017). The TCCON–NDACC-IRWG networks have been operating since 2004 and 1992 and provide time series of many atmospheric constituents, including GHGs such as H2O, HDO, CO2, CH4, CH3D, N2O, SF6, O3, C2H6, CCl3F, CCl2F2, and CHClF2. For solar zenith angles (SZAs) of less than 80∘, the total errors of , , and are less than 0.25 % (∼1 ppmv), 0.5 % (∼5 ppbv), and 1 % (∼3 ppbv), respectively (Wunch et al., 2011). These observations have been extensively used in investigations of carbon cycle, carbon source and transport, satellite validation, development of remote sensing algorithm, and evaluation of atmospheric CTMs. Currently, there are only ∼30 TCCON–NDACC-IRWG joint stations around the globe, most of them distributed in Europe and North America, and the stations in other parts of the globe is sparse (Fig. 3). Currently, only two TCCON stations have been set up in China, the Hefei station (32.0∘ N, 117.2∘ E) and the Xianghe station (39.75∘ N, 116.96∘ E) (Tian et al., 2017; Wang et al., 2017; Y. Yang et al., 2020).
Despite their outstanding capabilities such as high precision and stability, the high-resolution IFS120/125HR FTIR spectrometers also have their limitations. They are expensive and ponderous spectrometers; their operation relies on a large number of infrastructure, and the maintenance for their optical alignments is difficult and time consuming. In order to address these issues, the usage of cheaper, smaller, and more transportable FTIR spectrometers has been investigated in recent years. These FTIR spectrometers including EM27/SUN and VERTEX-80/SUN manufactured by Bruker, Germany, have been verified to have capacity comparable to the IFS125HR with respect to GHG monitoring. The transportability of the EM27/SUN and VERTEX-80/SUN spectrometers favors campaign use, and many successful campaigns were conducted by various scientists (Hase et al., 2015; Hedelius et al., 2016; Frey et al., 2019, 2021; Vogel et al., 2019; Ars et al., 2020; Jacobs et al., 2020; Tu et al., 2020; Mermigkas et al., 2021). Generally, scientists first use the high accuracy of the high-resolution FTIR dataset to calibrate the EM27/SUN and VERTEX-80/SUN spectrometers, and then they use the transportable spectrometers to derive the emission rate of a city, an industrial facility, or a landfill. With the transportable EM27/SUN spectrometers, the COllaborative Carbon Column Observing Network (COCCON) has been built to derive column-averaged abundances of GHGs over the world (Hase et al., 2015; Frey et al., 2019). The EM27/SUN and VERTEX-80/SUN observations can complement the high-resolution FTIR observations around the globe (Table S2).
In addition to the network-based routine observations, there are also many research-oriented GHG campaigns around the globe, and these uncoordinated campaigns are too numerous to count accurately (Gerbig et al., 2003; Lin et al., 2006; Zellweger et al., 2016; Gomez-Pelaez et al., 2019; Liu et al., 2021a, b). These GHG measurements are made using CRDS, NDIR spectroscopy, off-axis integrated cavity output spectroscopy (OA-ICOS), gas chromatography with flame ionization detection (GC/FID), FTIR spectroscopy, or differential absorption lidar (DIAL) implemented on different platforms (Krings et al., 2011, 2013, 2018; Zellweger et al., 2016; Krautwurst et al., 2017, 2021). For example, the airborne MAMAP (Methane Airborne Mapper) spectrometer developed by the University of Bremen can be used to derive point source rates of CH4 and CO2 (Krings et al., 2011, 2013, 2018). Japanese scientists have developed a grating-based optical spectrum analyzer (OSA) and an optical fiber Fabry–Pérot interferometer (FFPI) to measure atmospheric CO2 and CH4 total columns (Kobayashi et al., 2010).
Overall, the international community has established a series of monitoring networks to measure GHGs on different spatiotemporal scales. Taking advantage of the fact that all GHGs have spectral absorptions in the infrared waveband, most of these networks are established by means of various spectroscopic instruments. These stereoscopic monitoring networks combining the emission inventory compilation and CTMs have formed a state-of-the-art GHG monitoring and assessment system (Zellweger et al., 2016; Krautwurst et al., 2021), which is extensively used by the United Nations Framework Convention on Climate Change (UNFCCC) to assess GHG emissions on global, national, and regional scales and identify who, which emission sector, and what quantity are responsible for respective GHG emissions (IPCC, 2019).
Global energy consumption data disclose that China overtook the United States in 2006 as the world's top CO2 producer, i.e., the biggest anthropogenic contributor to global warming (IPCC, 2019). The severity, extension, complexity, and the need-to-cut scale of GHG emissions in China are unrivaled compared to other countries (Liu et al., 2022). Facing one of the most serious climate change problems around the globe, China has to address a series of scientific, technical, and management issues to achieve the goals of carbon peak/neutrality. As China pays more and more attention to climate change, the Chinese government has put a large effort into the development of GHG monitoring capacity. Although efforts to monitor GHGs in China have hitherto been largely uncoordinated with the established international networks, the GHG monitoring capacity has been steadily improved. Chinese scientists have conducted many GHG monitoring studies in urban agglomerations or typical industrial parks in different city clusters such as the Yangtze River Delta (YRD), North China Plain (NCP), and Pearl River Delta (PRD) (Tian et al., 2017, 2018; Wang et al., 2019; Li et al., 2021; Liu et al., 2021b); in background areas such as Waliguan in Qinghai Province and Longfengshan in Heilongjiang Province (Fang et al., 2014, 2015a, 2016); and in offshore areas such as the South China Sea, Yellow Sea, and Bohai Bay (Gerbig et al., 2003; Liu et al., 2021a, b). Research institutions, monitoring technologies, monitoring platforms, monitoring scales, monitoring applications, and typical advances can be summarized in Table 1. We elaborate this as follows.
-
Monitoring technologies include a variety of active and passive measurement technologies, which mainly include electrochemical (EC) sensing technology, tunable diode laser absorption spectroscopy (TDLAS), differential optical absorption spectroscopy (DOAS), FTIR, NDIR, GC/FID, lidar, CRDS, OA-ICOS, photoacoustic spectroscopy (PAS), etc. All these techniques can be classified as spectroscopic technique except EC technology, which uses capacitive readout cantilevers to detect an absorbed signal (Zellweger et al., 2016; Liu et al., 2022). In most cases, the EC, TDLAS, NDIR, GC/FID, lidar, CRDS, OA-ICOS, and PAS techniques are commonly used for active measurement platforms, while DOAS and FTIR techniques are extensively used for both active and passive measurement platforms. Figure 4 illustrates the principles of spectroscopic techniques for GHG monitoring. The active measurement techniques (a, f) use artificial light sources and the passive measurement techniques (b–e) use natural light sources such as sunlight to monitor GHGs. For both active and passive measurement techniques, the absorbed signals can be detected from direct transmission (a, b), surface reflection (e), or atmospheric scattering (c, d, f). Currently, the spectroscopic technique is the only technology that can be used to observe global GHGs from space.
-
Monitoring platforms include manual sampling analysis, surface in situ measurement sites (e.g., laboratory measurement or surface monitoring network); ground-based remote sensing platforms (such as ground-based FTIR, lidar, and DOAS observatories); and tower-based, airborne, space-based (e.g., GF-5 series, TanSat satellites, and space-borne lidar), ship-borne, vehicle-borne, unmanned aerial vehicle (UAV), balloon, tethered balloon, and other monitoring platforms. Since any single monitoring platform cannot fully meet the requirements of stereoscopic monitoring of GHG emissions due to its limited coverage or spatial resolution, scientists usually integrate a suite of observation platforms to form a stereoscopic monitoring system (Fig. 5). However, most stereoscopic GHG monitoring activities in China have been research-oriented, temporal, sparse, and uncoordinated with the established international networks (Fang et al., 2014; Tian et al., 2017, 2018; Wang et al., 2017; Y. Yang et al., 2020; Liu et al., 2021a, b; Sun et al., 2021).
-
The monitoring spatial scale ranges from single point sources, single constituents, small scale, and regional scale to clustered multi-point sources, multiple constituents, large scale, and global scale (Zellweger et al., 2016). Depending on monitoring technologies, constituents and platforms, monitoring temporal resolution ranges from seconds to minutes, hours, and days. Monitoring spatial resolution ranges from a meter to dozens of meters and a kilometer to dozens of kilometers. Monitoring accuracy ranges from the thousandth level to percent level, and monitoring sensitivity ranges from the parts per billion by volume to parts per million by volume level. Usually the more abundant GHGs tend to produce stronger spectroscopic absorptions, which makes them easier to be separated from background and thus can be monitored with high sensitivity. Although traditional EC or manual sampling analysis techniques are capable of measuring many GHGs with satisfactory accuracy, they usually have limited coverage and can only measure one constituent at a time. In comparison, spectroscopic technology can have a larger coverage, wider monitoring range, and more sensitivity and can continuously real-time monitor multiple constituents at a time. In particular, a single spectroscopic instrument can simultaneously monitor several GHGs without disturbing the samples; i.e., the monitoring process can be completely unattended. As long as an appropriate waveband is selected, the volume mixing ratio (VMR) concentrations of some GHGs can be measured with a sensitivity of less than 1 ppmv. The coverage can be extended from several meters to several kilometers without multi-point sampling.
-
Monitoring targets include H2O, HDO, CO2, CH4, CH3D, N2O, SF6, O3, C2H6, CCl3F, CCl2F2, and CHClF2 (Sun et al., 2018b). Monitoring regions include typical industrial zones, industrial stack emissions, urban atmosphere, ambient atmosphere, remote background regions, offshore regions, wetlands, etc. These GHG measurements with different spatiotemporal scales have been extensively used in investigations of the global carbon cycle, GHG trends, regional GHG sources and transport, ecological GHG flux estimate, urban or industrial GHG emissions estimates, validations of CTMs and emission inventory, multi-platform cross calibration, and algorithm improvement (De Maziere et al., 2018; Tian et al., 2018; Sun et al., 2021).
With decades of effort, China has made a great breakthrough in GHG monitoring capacity and steadily improved the performance of homemade GHG monitoring instruments. Typical advances in GHG monitoring in China include, but are not limited to, the following aspects.
-
The TanSat and GF-5 series GHG satellite payloads developed by China have successfully obtained high precision of global CO2 distributions (Li et al., 2016; Wu et al., 2018; X. Y. Zhang et al., 2020; Zhao et al., 2021; Cai et al., 2022). Comparisons with the TCCON, GOSAT, and OCO-2 data show that some key performance indicators such as accuracy, precision, and spatiotemporal resolution of these Chinese GHG satellites have reached the envisaged requirements (Liu et al., 2013, 2014, 2018; Cai et al., 2014; Du et al., 2018; D. X. Yang et al., 2018; Z. D. Yang et al., 2018b; Li et al., 2019; Zhang et al., 2019; Zhao et al., 2021; Bao et al., 2020; S. P. Wang et al., 2020; D. Yang et al., 2020; Boesch et al., 2021; Yang et al., 2021a, b). Both TanSat and GF-5 series GHG payloads use passive remote sensing technology to derive global CO2 distributions from scattered sunlight. As a result, they can only work in the daytime and are also seriously influenced by clouds and aerosols. The first Chinese space-borne CO2 lidar on board the atmospheric environment monitoring satellite launched on 16 April 2022 used active remote sensing technology to derive global CO2 distributions. Its operation does not rely on sunlight and is less influenced by clouds and aerosols, which will greatly improve the global CO2 mapping capacity.
-
A series of in situ online, ground-based, and airborne instruments have been developed by Chinese scientists to investigate the diurnal, monthly, seasonal, and inter-annual variabilities and spatial distributions of key GHGs (Tang et al., 2006); speculate on their sources and sinks; and reveal the physical and chemical mechanisms that drive their variabilities. For example, Chinese scientists have developed a suite of in situ spectroscopic instruments to measure surface VMRs and isotope ratios of GHGs in background atmosphere, sea–air CO2 flux in the coastal ocean boundary layer, and soil–air CO2 flux in farmland (Gerbig et al., 2003; Liu et al., 2021a, b). They have also developed a suite of ground-based spectroscopic instruments for measuring total columns of GHGs (Tian et al., 2018), vehicle-based spectroscopic instruments for industrial GHG emissions, and airborne spectroscopic instruments for deriving the spatial distributions of CO2 in the North China Plain (Wang et al., 2019; Shi et al., 2021).
-
The ground-based high-resolution FTIR observatory at Hefei has continuously observed the total columns or profiles of H2O, HDO, CO2, CH4, CH3D, N2O, SF6, O3, C2H6, CCl3F, CCl2F2, and CHClF2 in eastern China since 2014 and has become a national infrastructure for ground-based validation of GF-5 series GHG satellites and other space-borne instruments (Sun et al., 2018a, b). The ground-based FTIR measurements at the Hefei observatory meet the TCCON quality requirements, and this station was formally accepted as a TCCON site in 2018. Ground-based FTIR CO2 measurements at the Hefei observatory showed an increasing change rate of (2.71±0.32) % per year between 2015 and 2019 (Fig. 6). A similar ground-based high-resolution FTIR observatory at Xianghe also passed the TCCON quality inspection and joined the TCCON network in 2021 (Y. Yang et al., 2020). Furthermore, a few affiliations started to operate the portable EM27/SUN FTIR spectrometers and became COCCON members in the last 3 years (Frey et al., 2019; Liu et al., 2022; Che et al., 2022a, b; Cai et al., 2021).
-
Some Chinese scientists have used commercial in situ instruments such as Picarro or Licor series GHG analyzers to investigate the spatiotemporal variabilities and emission flux of GHGs in different regions of China (Lin et al., 2006; Tang et al., 2006; Fang et al., 2016; Tian et al., 2018; Yi et al., 2019; Li et al., 2021; Liu et al., 2021b). With the publicly accessible OCO-2 satellite data, Chinese scientists have estimated CO2 anthropogenic emissions of cities and industrial regions in China. The satellite-based CO2 emissions are generally in good agreement with the MEIC emission inventory values but are more different from the global gridded EDGAR and ODIAC emission datasets (Zheng et al., 2020a, b). Most recently, Chinese scientists have used the publicly accessible OCO-2 satellite observations to quantify CO2 emissions down to individual point sources such as middle- to large-sized coal power plants over China (Zheng et al., 2020a; Hu and Shi, 2021).
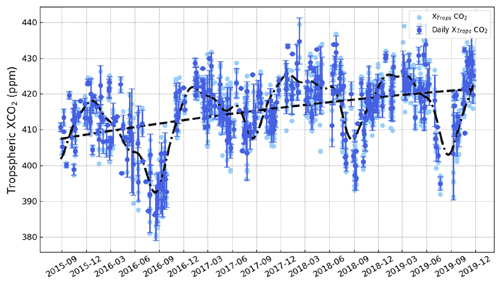
Figure 6Ground-based FTIR CO2 measurements at the Hefei observatory (Shan et al., 2021). The seasonality and interannual variability are represented by the black dashed curve and black dashed line, respectively, which are fitted by using a bootstrap resampling model with a third Fourier series plus a linear function.
Governments around the globe are committed to providing credible data to support the global carbon budget, which promotes the emergence of the state-of-the-art GHG monitoring technology in developed countries. As the world's top CO2 producer, China faces both challenges and opportunities. One of the major challenges is how we can accurately monitor GHG emissions under the complex carbon emission scenarios over China. GHG emissions in China are complex and diverse (Liu et al., 2022). GHG concentrations measured at a specific place include both local generation and long-range transport, which occurs not only near the surface but also in the upper atmosphere. In addition, China has a complex ecological environment characterized by high aerosol levels, high variability, and compound pollution mixed with many constituents, which poses unprecedented challenges (i.e., increase monitoring uncertainty) to the establishment of a GHG stereoscopic monitoring network in China. Fortunately, China can learn from other countries (IPCC, 2019). Through in-depth cooperation with the international community, China can establish a reliable GHG monitoring network with international credibility.
Accurate knowledge of regional GHG emissions requires accurate measurements of GHG variabilities on different spatial scales, including the in situ “point” concentrations reflecting the small-scale level, the “column” concentration reflecting the mesoscale level, and more importantly the “profile” concentration reflecting vertical distribution of GHGs. In order to develop a creditable GHG stereoscopic monitoring network in China, some key technical questions need to be solved, which are summarized as follows.
-
In terms of specific in situ monitoring and remote sensing technologies, how can different monitoring technologies and monitoring platforms learn from and complement each other by means of intensive comparison, verification, and optimization?
-
In terms of organization and implementation, how can we take full advantage of various monitoring platforms and technologies, make full use of their strengths and avoid their weaknesses, and make concerted efforts to achieve stereoscopic GHG monitoring for specific carbon source and carbon sink scenarios?
-
In terms of data fusion, how can we assimilate multiple datasets collected from different platforms and technologies to generate a new uniform dataset that has better coverage than the original dataset without reducing their accuracy, which would improve our understanding of carbon cycle mechanisms and promote the development of a GHG forecasting model?
Although much of China's economic growth still relies on the high-carbon energy previously implemented by developed countries, China is committed to achieving the goals of carbon peak by 2030 and carbon neutrality by 2060. In order to support the formulation of low-carbon policies for achieving the goals of carbon peak/neutrality, China should improve its GHG monitoring capability as soon as possible. It is suggested to take full advantage of various monitoring technologies, monitoring platforms, numerical simulations, and inventory compilation techniques to form a creditable GHG stereoscopic monitoring and assessment (M&A) system. Implementation of this M&A system should be coordinated with the established international networks and routinely quantify GHGs on global, national, provincial, regional, and individual point scales with high spatiotemporal resolution and wide coverage. Improved knowledge of carbon emissions on different scales is very useful for adjustment of low-carbon policy in China. In view of status, advances, and challenges of China's GHG monitoring, future developments are expected to focus on the following aspects.
-
The development of high-end GHG monitoring technology, instruments, and core components should be strengthened to improve GHG monitoring capacity in China. Development priorities include intelligent and miniaturized instruments dedicated for profile and flux of GHGs within multi-sphere ecological environment and key optical components such as high-resolution spectrometer, light source, solid laser, high-reflectivity mirror, narrow-band filter, detector, etc. It is expected that homemade GHG monitoring instruments can meet routine GHG monitoring demands in China in the near future.
-
It is suggested to routinely monitor GHG over typical GHGs sources and atmospheric background regions, which favor the verification of a GHG emission inventory and the implement of nationwide carbon trading. At present, the number of GHG monitoring sites in China remains sparse. The rural regions are rarely covered and there are only a few monitoring stations located in western China. GHGs are not included in the atmospheric constituents routinely monitored by the China National Environmental Monitoring Center (CNEMC) network. It is suggested to include key GHGs in China's surface environmental quality monitoring network, which will improve China's GHG monitoring capacity.
-
Since roughly 70 % of the Earth is shrouded by clouds at any given moment and GHG monitoring from space is prone to cloud interference, a single satellite can only provide a small number of observations per year suitable for emission estimates for any given GHG source. It is necessary to routinely monitor GHGs over China with satellite constellations, which can offer better spatiotemporal resolution and coverage compared to a single satellite alone. Only with high spatiotemporal resolution and coverage can we routinely quantify GHGs on global, national, provincial, regional, and individual point scales to support adjustment of low-carbon policy.
-
Monitoring data quality control, multi-source data fusion, and data-sharing platforms should be systematized and standardized. By standardizing data quality control and data fusion technology of multi-source GHG metadata and establishing a systematic data-sharing mechanism, the metadata can eventually be applied in carbon reduction governance and decision-making by management departments.
-
It is suggested to establish an inter-departmental management agency for GHG monitoring in China, where the government serves as the leader, and the technology holders and expert communities are participating. Furthermore, it is of great significance to unite the environmental protection industry association of China, Chinese association of environmental science, research institutions, universities, enterprises, and other professional communities to build a uniform verification standard for GHG monitoring, which should be in cooperation with international networks/partners to achieve common global criteria. This verification standard can not only standardize GHG monitoring technology but also disclose its verification criteria and process, which can promote the implementation of new GHG monitoring technology in China.
GHG monitoring capability in China has achieved rapid improvement in recent years. Relying on homemade technologies and instruments, combined with publicly accessible space-borne observation instruments and open-source remote sensing algorithms, China has conducted a suite of GHG stereoscopic monitoring studies in different regions of China, but most of them have been research-oriented, temporal, sparse, and uncoordinated with the established international networks. Some key technical indicators such as spatiotemporal resolution, coverage, and accuracy need to be further improved. Furthermore, monitoring data quality control, multi-source data fusion, and data-sharing platforms have not been standardized.
In order to support the formulation of green economic policies for achieving the goals of carbon peak/neutrality, China should improve its GHG monitoring capability soon. It is suggested to take full advantage of various monitoring technologies, monitoring platforms, numerical simulations, and inventory compilation techniques to form a creditable GHG stereoscopic monitoring and assessment (M&A) system. Implementation of this M&A system should be coordinated with the established international networks and routinely quantify GHGs on global, national, provincial, regional, and individual point scales with high spatiotemporal resolution and wide coverage. Improved knowledge of carbon emissions on different scales is very useful for adjustment of low-carbon policy in China.
The geolocations of all sites coordinated by NOAA and WMO and all FTIR sites coordinated by TCCON, NDACC-IRWG, and COCCON are summarized in the Supplement. All other data are available from Youwen Sun upon request (ywsun@aiofm.ac.cn).
The supplement related to this article is available online at: https://doi.org/10.5194/amt-15-4819-2022-supplement.
CL designed the study. YS wrote the paper. HY, WW, CS, JN, MP, KL, and ZC improved the content.
The contact author has declared that none of the authors has any competing interests.
Publisher's note: Copernicus Publications remains neutral with regard to jurisdictional claims in published maps and institutional affiliations.
This work is supported by the Youth Innovation Promotion Association, CAS (no. 2019434).
This research has been supported by the Youth Innovation Promotion Association, CAS (grant no. 2019434), and the Sino-German Mobility program (grant no. M-0036).
This paper was edited by Keding Lu and reviewed by two anonymous referees.
Ars, S., Vogel, F., Arrowsmith, C., Heerah, S., Knuckey, E., Lavoie, J., Lee, C., Pak, N. M., Phillips, J. L., and Wunch, D.: Investigation of the Spatial Distribution of Methane Sources in the Greater Toronto Area Using Mobile Gas Monitoring Systems, Environ. Sci. Technol., 54, 15671–15679, 2020.
Bao, Z. Y., Zhang, X. Y., Yue, T. X., Zhang, L. L., Wang, Z., Jiao, Y. M., Bai, W. G., and Meng, X. Y.: Retrieval and Validation of XCO2 from TanSat Target Mode Observations in Beijing, Remote Sens.-Basel, 12, 3063, 2020.
Barré, J., Aben, I., Agustí-Panareda, A., Balsamo, G., Bousserez, N., Dueben, P., Engelen, R., Inness, A., Lorente, A., McNorton, J., Peuch, V.-H., Radnoti, G., and Ribas, R.: Systematic detection of local CH4 anomalies by combining satellite measurements with high-resolution forecasts, Atmos. Chem. Phys., 21, 5117–5136, https://doi.org/10.5194/acp-21-5117-2021, 2021.
Belikov, D. A., Maksyutov, S., Ganshin, A., Zhuravlev, R., Deutscher, N. M., Wunch, D., Feist, D. G., Morino, I., Parker, R. J., Strong, K., Yoshida, Y., Bril, A., Oshchepkov, S., Boesch, H., Dubey, M. K., Griffith, D., Hewson, W., Kivi, R., Mendonca, J., Notholt, J., Schneider, M., Sussmann, R., Velazco, V. A., and Aoki, S.: Study of the footprints of short-term variation in XCO2 observed by TCCON sites using NIES and FLEXPART atmospheric transport models, Atmos. Chem. Phys., 17, 143–157, https://doi.org/10.5194/acp-17-143-2017, 2017.
Boesch, H., Liu, Y., Tamminen, J., Yang, D. X., Palmer, P. I., Lindqvist, H., Cai, Z. N., Che, K., Di Noia, A., Feng, L., Hakkarainen, J., Ialongo, I., Kalaitzi, N., Karppinen, T., Kivi, R., Kivimaki, E., Parker, R. J., Preval, S., Wang, J., Webb, A. J., Yao, L., and Chen, H. L.: Monitoring Greenhouse Gases from Space, Remote Sens.-Basel, 13, 2700, 2021.
Buchwitz, M., Reuter, M., Schneising, O., Boesch, H., Guerlet, S., Dils, B., Aben, I., Armante, R., Bergamaschi, P., Blumenstock, T., Bovensmann, H., Brunner, D., Buchmann, B., Burrows, J. P., Butz, A., Chedin, A., Chevallier, F., Crevoisier, C. D., Deutscher, N. M., Frankenberg, C., Hase, F., Hasekamp, O. P., Heymann, J., Kaminski, T., Laeng, A., Lichtenberg, G., De Maziere, M., Noel, S., Notholt, J., Orphal, J., Popp, C., Parker, R., Scholze, M., Sussmann, R., Stiller, G. P., Warneke, T., Zehner, C., Bril, A., Crisp, D., Griffith, D. W. T., Kuze, A., O'Dell, C., Oshchepkov, S., Sherlock, V., Suto, H., Wennberg, P., Wunch, D., Yokota, T., and Yoshida, Y.: The Greenhouse Gas Climate Change Initiative (GHG-CCI): Comparison and quality assessment of near-surface-sensitive satellite-derived CO2 and CH4 global data sets, Remote Sens. Environ., 162, 344–362, 2015.
Butz, A., Guerlet, S., Hasekamp, O., Schepers, D., Galli, A., Aben, I., Frankenberg, C., Hartmann, J. M., Tran, H., Kuze, A., Keppel-Aleks, G., Toon, G., Wunch, D., Wennberg, P., Deutscher, N., Griffith, D., Macatangay, R., Messerschmidt, J., Notholt, J., and Warneke, T.: Toward accurate CO2 and CH4 observations from GOSAT, Geophys. Res. Lett., 38, L14812, https://doi.org/10.1029/2011GL047888, 2011.
Butz, A., Galli, A., Hasekamp, O., Landgraf, J., Tol, P., and Aben, I.: TROPOMI aboard Sentinel-5 Precursor: Prospective performance of CH4 retrievals for aerosol and cirrus loaded atmospheres, Remote Sens. Environ., 120, 267–276, 2012.
Cai, Z., Che, K., Liu, Y., Yang, D., Liu, C., and Yue, X.: Decreased Anthropogenic CO2 Emissions during the COVID-19 Pandemic Estimated from FTS and MAX-DOAS Measurements at Urban Beijing, Remote Sens.-Basel, 13, 517, https://doi.org/10.3390/rs13030517, 2021.
Cai, Z., Sun, K., Yang, D., Liu, Y., Yao, L., Lin, C., and Liu, X.: On-Orbit Characterization of TanSat Instrument Line Shape Using Observed Solar Spectra, Remote Sens.-Basel, 14, 3334, https://doi.org/10.3390/rs14143334, 2022.
Cai, Z. N., Liu, Y., and Yang, D. X.: Analysis of XCO2 retrieval sensitivity using simulated Chinese Carbon Satellite (TanSat) measurements, Sci. China Earth Sci., 57, 1919–1928, 2014.
Che, K., Cai, Z., Liu, Y., Wu, L., Yang, D., Chen, Y., Meng, X., Zhou, M., Wang, J., Yao, L., and Wang, P.: Lagrangian inversion of anthropogenic CO2 emissions from Beijing using differential column measurements, Environ. Res. Lett., 17, 075001, https://doi.org/10.1088/1748-9326/ac7477, 2022a.
Che, K., Liu, Y., Cai, Z. N., Yang, D. X., Wang, H. B., Ji, D. H., Yang, Y., and Wang, P. C.: Characterization of regional combustion efficiency using ΔXCO:ΔXCO2 observed by a portable Fourier Transform spectrometer at an urban site in Beijing, 5, 1299–1315, https://doi.org/10.1007/s00376-022-1247-7, 2022b.
Chevallier, F., Deutscher, N. M., Conway, T. J., Ciais, P., Ciattaglia, L., Dohe, S., Frohlich, M., Gomez-Pelaez, A. J., Griffith, D., Hase, F., Haszpra, L., Krummel, P., Kyro, E., Labuschagne, C., Langenfelds, R., Machida, T., Maignan, F., Matsueda, H., Morino, I., Notholt, J., Ramonet, M., Sawa, Y., Schmidt, M., Sherlock, V., Steele, P., Strong, K., Sussmann, R., Wennberg, P., Wofsy, S., Worthy, D., Wunch, D., and Zimnoch, M.: Global CO2 fluxes inferred from surface air-sample measurements and from TCCON retrievals of the CO2 total column, Geophys. Res. Lett., 38, L24810, https://doi.org/10.1029/2011GL049899, 2011.
Cogan, A. J., Boesch, H., Parker, R. J., Feng, L., Palmer, P. I., Blavier, J. F. L., Deutscher, N. M., Macatangay, R., Notholt, J., Roehl, C., Warneke, T., and Wunch, D.: Atmospheric carbon dioxide retrieved from the Greenhouse gases Observing SATellite (GOSAT): Comparison with ground-based TCCON observations and GEOS-Chem model calculations, J. Geophys. Res.-Atmos., 117, D21301, https://doi.org/10.1029/2012JD018087, 2012.
Connor, B. J., Sherlock, V., Toon, G., Wunch, D., and Wennberg, P. O.: GFIT2: an experimental algorithm for vertical profile retrieval from near-IR spectra, Atmos. Meas. Tech., 9, 3513–3525, https://doi.org/10.5194/amt-9-3513-2016, 2016.
De Mazière, M., Thompson, A. M., Kurylo, M. J., Wild, J. D., Bernhard, G., Blumenstock, T., Braathen, G. O., Hannigan, J. W., Lambert, J.-C., Leblanc, T., McGee, T. J., Nedoluha, G., Petropavlovskikh, I., Seckmeyer, G., Simon, P. C., Steinbrecht, W., and Strahan, S. E.: The Network for the Detection of Atmospheric Composition Change (NDACC): history, status and perspectives, Atmos. Chem. Phys., 18, 4935–4964, https://doi.org/10.5194/acp-18-4935-2018, 2018.
Deng, F., Jones, D. B. A., Henze, D. K., Bousserez, N., Bowman, K. W., Fisher, J. B., Nassar, R., O'Dell, C., Wunch, D., Wennberg, P. O., Kort, E. A., Wofsy, S. C., Blumenstock, T., Deutscher, N. M., Griffith, D. W. T., Hase, F., Heikkinen, P., Sherlock, V., Strong, K., Sussmann, R., and Warneke, T.: Inferring regional sources and sinks of atmospheric CO2 from GOSAT XCO2 data, Atmos. Chem. Phys., 14, 3703–3727, https://doi.org/10.5194/acp-14-3703-2014, 2014.
Dils, B., Buchwitz, M., Reuter, M., Schneising, O., Boesch, H., Parker, R., Guerlet, S., Aben, I., Blumenstock, T., Burrows, J. P., Butz, A., Deutscher, N. M., Frankenberg, C., Hase, F., Hasekamp, O. P., Heymann, J., De Mazière, M., Notholt, J., Sussmann, R., Warneke, T., Griffith, D., Sherlock, V., and Wunch, D.: The Greenhouse Gas Climate Change Initiative (GHG-CCI): comparative validation of GHG-CCI SCIAMACHY/ENVISAT and TANSO-FTS/GOSAT CO2 and CH4 retrieval algorithm products with measurements from the TCCON, Atmos. Meas. Tech., 7, 1723–1744, https://doi.org/10.5194/amt-7-1723-2014, 2014.
Du, S. S., Liu, L. Y., Liu, X. J., Zhang, X., Zhang, X. Y., Bi, Y. M., and Zhang, L. C.: Retrieval of global terrestrial solar-induced chlorophyll fluorescence from TanSat satellite, Sci. Bull., 63, 1502–1512, 2018.
Eldering, A., Wennberg, P. O., Crisp, D., Schimel, D. S., Gunson, M. R., Chatterjee, A., Liu, J., Schwandner, F. M., Sun, Y., O'Dell, C. W., Frankenberg, C., Taylor, T., Fisher, B., Osterman, G. B., Wunch, D., Hakkarainen, J., Tamminen, J., and Weir, B.: The Orbiting Carbon Observatory-2 early science investigations of regional carbon dioxide fluxes, Science, 358, eaam5745, https://doi.org/10.1126/science.aam5745, 2017.
Fang, S. X., Zhou, L. X., Tans, P. P., Ciais, P., Steinbacher, M., Xu, L., and Luan, T.: In situ measurement of atmospheric CO2 at the four WMO/GAW stations in China, Atmos. Chem. Phys., 14, 2541–2554, https://doi.org/10.5194/acp-14-2541-2014, 2014.
Fang, S. X., Luan, T., Zhang, G., Wu, Y. L., and Yu, D. J.: The determination of regional CO2 mole fractions at the Longfengshan WMO/GAW station: A comparison of four data filtering approaches, Atmos. Environ., 116, 36–43, 2015a.
Fang, S. X., Tans, P. P., Steinbacher, M., Zhou, L. X., and Luan, T.: Comparison of the regional CO2 mole fraction filtering approaches at a WMO/GAW regional station in China, Atmos. Meas. Tech., 8, 5301–5313, https://doi.org/10.5194/amt-8-5301-2015, 2015b.
Fang, S. X., Tans, P. P., Steinbacher, M., Zhou, L. X., Luan, T., and Li, Z.: Observation of atmospheric CO2 and CO at Shangri-La station: results from the only regional station located at southwestern China, Tellus B, 68, 28506, 2016.
Frankenberg, C., Pollock, R., Lee, R. A. M., Rosenberg, R., Blavier, J.-F., Crisp, D., O'Dell, C. W., Osterman, G. B., Roehl, C., Wennberg, P. O., and Wunch, D.: The Orbiting Carbon Observatory (OCO-2): spectrometer performance evaluation using pre-launch direct sun measurements, Atmos. Meas. Tech., 8, 301–313, https://doi.org/10.5194/amt-8-301-2015, 2015.
Frey, M., Sha, M. K., Hase, F., Kiel, M., Blumenstock, T., Harig, R., Surawicz, G., Deutscher, N. M., Shiomi, K., Franklin, J. E., Bösch, H., Chen, J., Grutter, M., Ohyama, H., Sun, Y., Butz, A., Mengistu Tsidu, G., Ene, D., Wunch, D., Cao, Z., Garcia, O., Ramonet, M., Vogel, F., and Orphal, J.: Building the COllaborative Carbon Column Observing Network (COCCON): long-term stability and ensemble performance of the EM27/SUN Fourier transform spectrometer, Atmos. Meas. Tech., 12, 1513–1530, https://doi.org/10.5194/amt-12-1513-2019, 2019.
Frey, M. M., Hase, F., Blumenstock, T., Dubravica, D., Groß, J., Göttsche, F., Handjaba, M., Amadhila, P., Mushi, R., Morino, I., Shiomi, K., Sha, M. K., de Mazière, M., and Pollard, D. F.: Long-term column-averaged greenhouse gas observations using a COCCON spectrometer at the high-surface-albedo site in Gobabeb, Namibia, Atmos. Meas. Tech., 14, 5887–5911, https://doi.org/10.5194/amt-14-5887-2021, 2021.
Gerbig, C., Lin, J. C., Wofsy, S. C., Daube, B. C., Andrews, A. E., Stephens, B. B., Bakwin, P. S., and Grainger, C. A.: Toward constraining regional-scale fluxes of CO2 with atmospheric observations over a continent: 2. Analysis of COBRA data using a receptor-oriented framework, J. Geophys. Res.-Atmos., 108, 4756, 2003.
Gomez-Pelaez, A. J., Ramos, R., Cuevas, E., Gomez-Trueba, V., and Reyes, E.: Atmospheric CO2, CH4, and CO with the CRDS technique at the Izaña Global GAW station: instrumental tests, developments, and first measurement results, Atmos. Meas. Tech., 12, 2043–2066, https://doi.org/10.5194/amt-12-2043-2019, 2019.
Hase, F., Frey, M., Blumenstock, T., Groß, J., Kiel, M., Kohlhepp, R., Mengistu Tsidu, G., Schäfer, K., Sha, M. K., and Orphal, J.: Application of portable FTIR spectrometers for detecting greenhouse gas emissions of the major city Berlin, Atmos. Meas. Tech., 8, 3059–3068, https://doi.org/10.5194/amt-8-3059-2015, 2015.
Hedelius, J. K., Viatte, C., Wunch, D., Roehl, C. M., Toon, G. C., Chen, J., Jones, T., Wofsy, S. C., Franklin, J. E., Parker, H., Dubey, M. K., and Wennberg, P. O.: Assessment of errors and biases in retrievals of X, X, XCO, and X from a 0.5 cm−1 resolution solar-viewing spectrometer, Atmos. Meas. Tech., 9, 3527–3546, https://doi.org/10.5194/amt-9-3527-2016, 2016.
Heymann, J., Reuter, M., Hilker, M., Buchwitz, M., Schneising, O., Bovensmann, H., Burrows, J. P., Kuze, A., Suto, H., Deutscher, N. M., Dubey, M. K., Griffith, D. W. T., Hase, F., Kawakami, S., Kivi, R., Morino, I., Petri, C., Roehl, C., Schneider, M., Sherlock, V., Sussmann, R., Velazco, V. A., Warneke, T., and Wunch, D.: Consistent satellite XCO2 retrievals from SCIAMACHY and GOSAT using the BESD algorithm, Atmos. Meas. Tech., 8, 2961–2980, https://doi.org/10.5194/amt-8-2961-2015, 2015.
Houweling, S., Krol, M., Bergamaschi, P., Frankenberg, C., Dlugokencky, E. J., Morino, I., Notholt, J., Sherlock, V., Wunch, D., Beck, V., Gerbig, C., Chen, H., Kort, E. A., Röckmann, T., and Aben, I.: A multi-year methane inversion using SCIAMACHY, accounting for systematic errors using TCCON measurements, Atmos. Chem. Phys., 14, 3991–4012, https://doi.org/10.5194/acp-14-3991-2014, 2014.
Hu, Y. Q. and Shi, Y. S.: Estimating CO2 Emissions from Large Scale Coal-Fired Power Plants Using OCO-2 Observations and Emission Inventories, Atmosphere, 12, 811, 2021.
IPCC: 2019 Refinement to the 2006 IPCC Guidelines for National Greenhouse Gas Inventories, edited by: Calvo Buendia, E., Tanabe, K., Kranjc, A., Jamsranjav, B., Fukuda, M., Ngarize, S., Osako, A., Pyrozhenko, Y., Shermanau, P., and Federici, S., Intergovernmental Panel on Climate Change, ISBN 978-4-88788-232-4, 2019.
Jacob, D. J., Varon, D. J., Cusworth, D. H., Dennison, P. E., Frankenberg, C., Gautam, R., Guanter, L., Kelley, J., McKeever, J., Ott, L. E., Poulter, B., Qu, Z., Thorpe, A. K., Worden, J. R., and Duren, R. M.: Quantifying methane emissions from the global scale down to point sources using satellite observations of atmospheric methane, Atmos. Chem. Phys., 22, 9617–9646, https://doi.org/10.5194/acp-22-9617-2022, 2022.
Jacobs, N., Simpson, W. R., Wunch, D., O'Dell, C. W., Osterman, G. B., Hase, F., Blumenstock, T., Tu, Q., Frey, M., Dubey, M. K., Parker, H. A., Kivi, R., and Heikkinen, P.: Quality controls, bias, and seasonality of CO2 columns in the boreal forest with Orbiting Carbon Observatory-2, Total Carbon Column Observing Network, and EM27/SUN measurements, Atmos. Meas. Tech., 13, 5033–5063, https://doi.org/10.5194/amt-13-5033-2020, 2020.
Jervis, D., McKeever, J., Durak, B. O. A., Sloan, J. J., Gains, D., Varon, D. J., Ramier, A., Strupler, M., and Tarrant, E.: The GHGSat-D imaging spectrometer, Atmos. Meas. Tech., 14, 2127–2140, https://doi.org/10.5194/amt-14-2127-2021, 2021.
Kiel, M., Wunch, D., Wennberg, P. O., Toon, G. C., Hase, F., and Blumenstock, T.: Improved retrieval of gas abundances from near-infrared solar FTIR spectra measured at the Karlsruhe TCCON station, Atmos. Meas. Tech., 9, 669–682, https://doi.org/10.5194/amt-9-669-2016, 2016.
Kiel, M., Eldering, A., Roten, D. D., Lin, J. C., Feng, S., Lei, R. X., Lauvaux, T., Oda, T., Roehl, C. M., Blavier, J. F., and Iraci, L. T.: Urban-focused satellite CO2 observations from the Orbiting Carbon Observatory-3: A first look at the Los Angeles megacity, Remote Sens. Environ., 258, 112314, https://doi.org/10.1016/j.rse.2021.112314, 2021.
Kobayashi, N., Inoue, G., Kawasaki, M., Yoshioka, H., Minomura, M., Murata, I., Nagahama, T., Matsumi, Y., Tanaka, T., Morino, I., and Ibuki, T.: Remotely operable compact instruments for measuring atmospheric CO2 and CH4 column densities at surface monitoring sites, Atmos. Meas. Tech., 3, 1103–1112, https://doi.org/10.5194/amt-3-1103-2010, 2010.
Krautwurst, S., Gerilowski, K., Jonsson, H. H., Thompson, D. R., Kolyer, R. W., Iraci, L. T., Thorpe, A. K., Horstjann, M., Eastwood, M., Leifer, I., Vigil, S. A., Krings, T., Borchardt, J., Buchwitz, M., Fladeland, M. M., Burrows, J. P., and Bovensmann, H.: Methane emissions from a Californian landfill, determined from airborne remote sensing and in situ measurements, Atmos. Meas. Tech., 10, 3429–3452, https://doi.org/10.5194/amt-10-3429-2017, 2017.
Krautwurst, S., Gerilowski, K., Borchardt, J., Wildmann, N., Gałkowski, M., Swolkień, J., Marshall, J., Fiehn, A., Roiger, A., Ruhtz, T., Gerbig, C., Necki, J., Burrows, J. P., Fix, A., and Bovensmann, H.: Quantification of CH4 coal mining emissions in Upper Silesia by passive airborne remote sensing observations with the Methane Airborne MAPper (MAMAP) instrument during the CO2 and Methane (CoMet) campaign, Atmos. Chem. Phys., 21, 17345–17371, https://doi.org/10.5194/acp-21-17345-2021, 2021.
Krings, T., Gerilowski, K., Buchwitz, M., Reuter, M., Tretner, A., Erzinger, J., Heinze, D., Pflüger, U., Burrows, J. P., and Bovensmann, H.: MAMAP – a new spectrometer system for column-averaged methane and carbon dioxide observations from aircraft: retrieval algorithm and first inversions for point source emission rates, Atmos. Meas. Tech., 4, 1735–1758, https://doi.org/10.5194/amt-4-1735-2011, 2011.
Krings, T., Gerilowski, K., Buchwitz, M., Hartmann, J., Sachs, T., Erzinger, J., Burrows, J. P., and Bovensmann, H.: Quantification of methane emission rates from coal mine ventilation shafts using airborne remote sensing data, Atmos. Meas. Tech., 6, 151–166, https://doi.org/10.5194/amt-6-151-2013, 2013.
Krings, T., Neininger, B., Gerilowski, K., Krautwurst, S., Buchwitz, M., Burrows, J. P., Lindemann, C., Ruhtz, T., Schüttemeyer, D., and Bovensmann, H.: Airborne remote sensing and in situ measurements of atmospheric CO2 to quantify point source emissions, Atmos. Meas. Tech., 11, 721–739, https://doi.org/10.5194/amt-11-721-2018, 2018.
Kuai, L., Wunch, D., Shia, R. L., Connor, B., Miller, C., and Yung, Y.: Vertically constrained CO2 retrievals from TCCON measurements, J. Quant. Spectrosc. Ra., 113, 1753–1761, 2012.
Kulawik, S., Wunch, D., O'Dell, C., Frankenberg, C., Reuter, M., Oda, T., Chevallier, F., Sherlock, V., Buchwitz, M., Osterman, G., Miller, C. E., Wennberg, P. O., Griffith, D., Morino, I., Dubey, M. K., Deutscher, N. M., Notholt, J., Hase, F., Warneke, T., Sussmann, R., Robinson, J., Strong, K., Schneider, M., De Mazière, M., Shiomi, K., Feist, D. G., Iraci, L. T., and Wolf, J.: Consistent evaluation of ACOS-GOSAT, BESD-SCIAMACHY, CarbonTracker, and MACC through comparisons to TCCON, Atmos. Meas. Tech., 9, 683–709, https://doi.org/10.5194/amt-9-683-2016, 2016.
Li, M., Feng, K. S., Zheng, H. R., Pan, C., Meng, J., Li, J. S., Guan, D. B., and Li, Y.: Supply chain effects of China's fast growing marine economy on greenhouse gas emissions, Environ. Res. Lett., 16, 054061, https://doi.org/10.1088/1748-9326/abf192, 2021.
Li, S., Li, C. L., Xu, S. Y., Zhang, H., and Zheng, Y. Q.: Preflight radiometric calibration of a carbon dioxide spectrometer, Meas. Sci. Technol., 30, 055401, https://doi.org/10.1088/1361-6501/ab0c6d, 2019.
Li, Y. F., Zhang, C. M., Liu, D. D., Chen, J., Rong, P., Zhang, X. Y., and Wang, S. P.: CO2 retrieval model and analysis in short-wave infrared spectrum, Optik, 127, 4422–4425, 2016.
Lin, J. C., Gerbig, C., Wofsy, S. C., Daube, B. C., Matross, D. M., Chow, V. Y., Gottlieb, E., Andrews, A. E., Pathmathevan, M., and Munger, J. W.: What have we learned from intensive atmospheric sampling field programmes of CO2?, Tellus B, 58, 331–343, 2006.
Liu, C., Sun, Y. W., Shan, C. G., Wang, W., Notholt, J., Palm, M., Yin, H., Tian, Y., Gao, J. X., and Mao, H. Q.: Long-term observations of atmospheric constituents at the first ground-based high resolution Fourier transform spectrometry observation station in China, Engineering, https://doi.org/10.1016/j.eng.2021.11.022, in press, 2022.
Liu, S., Fang, S., Liu, P., Liang, M., Guo, M., and Feng, Z.: Measurement report: Changing characteristics of atmospheric CH4 in the Tibetan Plateau: records from 1994 to 2019 at the Mount Waliguan station, Atmos. Chem. Phys., 21, 393–413, https://doi.org/10.5194/acp-21-393-2021, 2021a.
Liu, S., Feng, Z. Z., Lin, H. W., Liu, P., Liang, M., Qing, X. M., Xiong, H. Y., Qiu, S. S., Li, J. X., Jiang, K., Hong, H. X., and Fang, S. X.: Changes of Atmospheric CO2 in the Tibetan Plateau From 1994 to 2019, J. Geophys. Res.-Atmos., 126, e2021JD035299, https://doi.org/10.1029/2021JD035299, 2021b.
Liu, Y., Yang, D. X., and Cai, Z. N.: A retrieval algorithm for TanSat XCO2 observation: Retrieval experiments using GOSAT data, Chinese Sci. Bull., 58, 1520–1523, 2013.
Liu, Y., Cai, Z. N., Yang, D. X., Zheng, Y. Q., Duan, M. Z., and Lu, D. R.: Effects of spectral sampling rate and range of CO2 absorption bands on XCO2 retrieval from TanSat hyperspectral spectrometer, Chinese Sci. Bull., 59, 1485–1491, 2014.
Liu, Y., Wang, J., Yao, L., Chen, X., Cai, Z. N., Yang, D. X., Yin, Z. S., Gu, S. Y., Tian, L. F., Lu, N. M., and Lyu, D. R.: The TanSat mission: preliminary global observations, Sci. Bull., 63, 1200–1207, 2018.
MacFaul, L.: Monitoring greenhouse gases, B. Am. Meteorol. Soc., 88, 1171–1171, 2007.
Mermigkas, M., Topaloglou, C., Balis, D., Koukouli, M. E., Hase, F., Dubravica, D., Borsdorff, T., and Lorente, A.: FTIR Measurements of Greenhouse Gases over Thessaloniki, Greece in the Framework of COCCON and Comparison with S5P/TROPOMI Observations, Remote Sens.-Basel, 13, 3395, 2021.
Messerschmidt, J., Geibel, M. C., Blumenstock, T., Chen, H., Deutscher, N. M., Engel, A., Feist, D. G., Gerbig, C., Gisi, M., Hase, F., Katrynski, K., Kolle, O., Lavrič, J. V., Notholt, J., Palm, M., Ramonet, M., Rettinger, M., Schmidt, M., Sussmann, R., Toon, G. C., Truong, F., Warneke, T., Wennberg, P. O., Wunch, D., and Xueref-Remy, I.: Calibration of TCCON column-averaged CO2: the first aircraft campaign over European TCCON sites, Atmos. Chem. Phys., 11, 10765–10777, https://doi.org/10.5194/acp-11-10765-2011, 2011.
Morino, I., Uchino, O., Inoue, M., Yoshida, Y., Yokota, T., Wennberg, P. O., Toon, G. C., Wunch, D., Roehl, C. M., Notholt, J., Warneke, T., Messerschmidt, J., Griffith, D. W. T., Deutscher, N. M., Sherlock, V., Connor, B., Robinson, J., Sussmann, R., and Rettinger, M.: Preliminary validation of column-averaged volume mixing ratios of carbon dioxide and methane retrieved from GOSAT short-wavelength infrared spectra, Atmos. Meas. Tech., 4, 1061–1076, https://doi.org/10.5194/amt-4-1061-2011, 2011.
Nassar, R., Hill, T. G., McLinden, C. A., Wunch, D., Jones, D. B. A., and Crisp, D.: Quantifying CO2 Emissions From Individual Power Plants From Space, Geophys. Res. Lett., 44, 10045–10053, 2017.
Pandey, S., Gautam, R., Houweling, S., van der Gon, H. D., Sadavarte, P., Borsdorff, T., Hasekamp, O., Landgraf, J., Tol, P., van Kempen, T., Hoogeveen, R., van Hees, R., Hamburg, S. P., Maasakkers, J. D., and Aben, I.: Satellite observations reveal extreme methane leakage from a natural gas well blowout, P. Natl. Acad. Sci. USA, 116, 26376–26381, 2019.
Pandey, S., Houweling, S., Lorente, A., Borsdorff, T., Tsivlidou, M., Bloom, A. A., Poulter, B., Zhang, Z., and Aben, I.: Using satellite data to identify the methane emission controls of South Sudan's wetlands, Biogeosciences, 18, 557–572, https://doi.org/10.5194/bg-18-557-2021, 2021.
Park, H., Jeong, S., Park, H., Labzovskii, L. D., and Bowman, K. W.: An assessment of emission characteristics of Northern Hemisphere cities using spaceborne observations of CO2, CO, and NO2, Remote Sens. Environ., 254, 112246, https://doi.org/10.1016/j.rse.2020.112246, 2021.
Parker, R. J., Webb, A., Boesch, H., Somkuti, P., Barrio Guillo, R., Di Noia, A., Kalaitzi, N., Anand, J. S., Bergamaschi, P., Chevallier, F., Palmer, P. I., Feng, L., Deutscher, N. M., Feist, D. G., Griffith, D. W. T., Hase, F., Kivi, R., Morino, I., Notholt, J., Oh, Y.-S., Ohyama, H., Petri, C., Pollard, D. F., Roehl, C., Sha, M. K., Shiomi, K., Strong, K., Sussmann, R., Té, Y., Velazco, V. A., Warneke, T., Wennberg, P. O., and Wunch, D.: A decade of GOSAT Proxy satellite CH4 observations, Earth Syst. Sci. Data, 12, 3383–3412, https://doi.org/10.5194/essd-12-3383-2020, 2020.
Patra, P. K., Crisp, D., Kaiser, J. W., Wunch, D., Saeki, T., Ichii, K., Sekiya, T., Wennberg, P. O., Feist, D. G., Pollard, D. F., Griffith, D. W. T., Velazco, V. A., De Maziere, M., Sha, M. K., Roehl, C., Chatterjee, A., and Ishijima, K.: The Orbiting Carbon Observatory (OCO-2) tracks 2–3 peta-gram increase in carbon release to the atmosphere during the 2014–2016 El Nino, Sci. Rep.-UK, 7, 13567, 2017.
Qu, Z., Jacob, D. J., Shen, L., Lu, X., Zhang, Y., Scarpelli, T. R., Nesser, H., Sulprizio, M. P., Maasakkers, J. D., Bloom, A. A., Worden, J. R., Parker, R. J., and Delgado, A. L.: Global distribution of methane emissions: a comparative inverse analysis of observations from the TROPOMI and GOSAT satellite instruments, Atmos. Chem. Phys., 21, 14159–14175, https://doi.org/10.5194/acp-21-14159-2021, 2021.
Reuter, M., Bovensmann, H., Buchwitz, M., Burrows, J. P., Connor, B. J., Deutscher, N. M., Griffith, D. W., Heymann, J., Keppel-Aleks, G., Messerschmidt, J., Notholt, J., Petri, C., Robinson, J., Schneising, O., Sherlock, V., Velazco, V. A., Warneke, T., Wennberg, P. O., and Wunch, D.: Retrieval of atmospheric CO2 with enhanced accuracy and precision from SCIAMACHY: validation with FTS measurements and comparison with model results, J. Geophys. Res., 116, 1, 2011.
Saito, R., Patra, P. K., Deutscher, N., Wunch, D., Ishijima, K., Sherlock, V., Blumenstock, T., Dohe, S., Griffith, D., Hase, F., Heikkinen, P., Kyrö, E., Macatangay, R., Mendonca, J., Messerschmidt, J., Morino, I., Notholt, J., Rettinger, M., Strong, K., Sussmann, R., and Warneke, T.: Technical Note: Latitude-time variations of atmospheric column-average dry air mole fractions of CO2, CH4 and N2O, Atmos. Chem. Phys., 12, 7767–7777, https://doi.org/10.5194/acp-12-7767-2012, 2012.
Schneising, O., Bergamaschi, P., Bovensmann, H., Buchwitz, M., Burrows, J. P., Deutscher, N. M., Griffith, D. W. T., Heymann, J., Macatangay, R., Messerschmidt, J., Notholt, J., Rettinger, M., Reuter, M., Sussmann, R., Velazco, V. A., Warneke, T., Wennberg, P. O., and Wunch, D.: Atmospheric greenhouse gases retrieved from SCIAMACHY: comparison to ground-based FTS measurements and model results, Atmos. Chem. Phys., 12, 1527–1540, https://doi.org/10.5194/acp-12-1527-2012, 2012.
Sha, M. K., Langerock, B., Blavier, J.-F. L., Blumenstock, T., Borsdorff, T., Buschmann, M., Dehn, A., De Mazière, M., Deutscher, N. M., Feist, D. G., García, O. E., Griffith, D. W. T., Grutter, M., Hannigan, J. W., Hase, F., Heikkinen, P., Hermans, C., Iraci, L. T., Jeseck, P., Jones, N., Kivi, R., Kumps, N., Landgraf, J., Lorente, A., Mahieu, E., Makarova, M. V., Mellqvist, J., Metzger, J.-M., Morino, I., Nagahama, T., Notholt, J., Ohyama, H., Ortega, I., Palm, M., Petri, C., Pollard, D. F., Rettinger, M., Robinson, J., Roche, S., Roehl, C. M., Röhling, A. N., Rousogenous, C., Schneider, M., Shiomi, K., Smale, D., Stremme, W., Strong, K., Sussmann, R., Té, Y., Uchino, O., Velazco, V. A., Vigouroux, C., Vrekoussis, M., Wang, P., Warneke, T., Wizenberg, T., Wunch, D., Yamanouchi, S., Yang, Y., and Zhou, M.: Validation of methane and carbon monoxide from Sentinel-5 Precursor using TCCON and NDACC-IRWG stations, Atmos. Meas. Tech., 14, 6249–6304, https://doi.org/10.5194/amt-14-6249-2021, 2021.
Shan, C., Wang, W., Liu, C., Guo, Y., Xie, Y., Sun, Y., Hu, Q., Zhang, H., Yin, H., and Jones, N.: Retrieval of vertical profiles and tropospheric CO2 columns based on high-resolution FTIR over Hefei, China, Opt. Express, 29, 4958–4977, https://doi.org/10.1364/OE.411383, 2021.
Shen, L., Zavala-Araiza, D., Gautam, R., Omara, M., Scarpelli, T., Sheng, J. X., Sulprizio, M. P., Zhuang, J. W., Zhang, Y. Z., Qu, Z., Lu, X., Hamburg, S. P., and Jacob, D. J.: Unravelling a large methane emission discrepancy in Mexico using satellite observations, Remote Sens. Environ., 260, 112461, https://doi.org/10.1016/j.rse.2021.112461, 2021.
Shi, T. Q., Han, Z. Y., Gong, W., Ma, X., and Han, G.: High-precision methodology for quantifying gas point source emission, J. Clean. Prod., 320, 128672, https://doi.org/10.1016/j.jclepro.2021.128672, 2021.
Singh, H. B., Brune, W. H., Crawford, J. H., Jacob, D. J., and Russell, P. B.: Overview of the summer 2004 intercontinental chemical transport experiment – North America (INTEX-A), J. Geophys. Res.-Atmos., 111, D24S01, https://doi.org/10.1029/2006JD007905, 2006.
Sun, Y., Liu, C., Chan, K., Wang, W., Shan, C. G., Hu, Q., and Liu, J. G.: The Influence of Instrumental Line Shape Degradation on the Partial Columns of O3, CO, CH4 and N2O Derived from High-Resolution FTIR Spectrometry, Remote Sens.-Basel, 10, 2041, 2018a.
Sun, Y., Palm, M., Liu, C., Hase, F., Griffith, D., Weinzierl, C., Petri, C., Wang, W., and Notholt, J.: The influence of instrumental line shape degradation on NDACC gas retrievals: total column and profile, Atmos. Meas. Tech., 11, 2879–2896, https://doi.org/10.5194/amt-11-2879-2018, 2018b.
Sun, Y., Yin, H., Liu, C., Mahieu, E., Notholt, J., Té, Y., Lu, X., Palm, M., Wang, W., Shan, C., Hu, Q., Qin, M., Tian, Y., and Zheng, B.: The reduction in C2H6 from 2015 to 2020 over Hefei, eastern China, points to air quality improvement in China, Atmos. Chem. Phys., 21, 11759–11779, https://doi.org/10.5194/acp-21-11759-2021, 2021.
Tang, X. L., Liu, S. G., Zhou, G. Y., Zhang, D. Q., and Zhou, C. Y.: Soil-atmospheric exchange of CO2, CH4, and N2O in three subtropical forest ecosystems in southern China, Global Change Biol., 12, 546–560, 2006.
Thompson, D. R., Benner, D. C., Brown, L. R., Crisp, D., Devi, V. M., Jiang, Y. B., Natraj, V., Oyafuso, F., Sung, K., Wunch, D., Castano, R., and Miller, C. E.: Atmospheric validation of high accuracy CO2 absorption coefficients for the OCO-2 mission, J. Quant. Spectrosc. Ra., 113, 2265–2276, 2012.
Tian, Y., Sun, Y., Liu, C., Wang, W., Shan, C., Xu, X., and Hu, Q.: Characterisation of methane variability and trends from near-infrared solar spectra over Hefei, China, Atmos. Environ., 173, 198–209, 2017.
Tian, Y., Sun, Y. W., Liu, C., Xie, P. H., Chan, K., Xu, J., Wang, W., and Liu, J. G.: Characterization of urban CO2 column abundance with a portable low resolution spectrometer (PLRS): Comparisons with GOSAT and GEOS-Chem model data, Sci. Total Environ., 612, 1593–1609, 2018.
Tu, Q., Hase, F., Blumenstock, T., Kivi, R., Heikkinen, P., Sha, M. K., Raffalski, U., Landgraf, J., Lorente, A., Borsdorff, T., Chen, H., Dietrich, F., and Chen, J.: Intercomparison of atmospheric CO2 and CH4 abundances on regional scales in boreal areas using Copernicus Atmosphere Monitoring Service (CAMS) analysis, COllaborative Carbon Column Observing Network (COCCON) spectrometers, and Sentinel-5 Precursor satellite observations, Atmos. Meas. Tech., 13, 4751–4771, https://doi.org/10.5194/amt-13-4751-2020, 2020.
Varon, D. J., Mckeever, J., Jervis, D., Maasakkers, J. D., Pandey, S., Houweling, S., Aben, I., Scarpelli, T., and Jacob, D. J.: Satellite Discovery of Anomalously Large Methane Point Sources From Oil/Gas Production, Geophys. Res. Lett., 46, 13507–13516, 2019.
Veefkind, J. P., Aben, I., Mcmullan, K., Forster, H., De Vries, J., Otter, G., Claas, J., Eskes, H. J., De Haan, J. F., Kleipool, Q., Van Weele, M., Hasekamp, O., Hoogeveen, R., Landgraf, J., Snel, R., Tol, P., Ingmann, P., Voors, R., Kruizinga, B., Vink, R., Visser, H., and Levelt, P. F.: TROPOMI on the ESA Sentinel-5 Precursor: A GMES mission for global observations of the atmospheric composition for climate, air quality and ozone layer applications, Remote Sens. Environ., 120, 70–83, 2012.
Vogel, F. R., Frey, M., Staufer, J., Hase, F., Broquet, G., Xueref-Remy, I., Chevallier, F., Ciais, P., Sha, M. K., Chelin, P., Jeseck, P., Janssen, C., Té, Y., Groß, J., Blumenstock, T., Tu, Q., and Orphal, J.: XCO2 in an emission hot-spot region: the COCCON Paris campaign 2015, Atmos. Chem. Phys., 19, 3271–3285, https://doi.org/10.5194/acp-19-3271-2019, 2019.
Wang, C., Guan, K. Y., Peng, B., Chen, M., Jiang, C. Y., Zeng, Y. L., Wu, G. H., Wang, S., Wu, J., Yang, X., Frankenberg, C., Kohler, P., Berry, J., Bernacchi, C., Zhu, K., Alden, C., and Miao, G. F.: Satellite footprint data from OCO-2 and TROPOMI reveal significant spatio-temporal and inter-vegetation type variabilities of solar-induced fluorescence yield in the US Midwest, Remote Sens. Environ., 241, 111728, https://doi.org/10.1016/j.rse.2020.111728, 2020.
Wang, R. W., Xie, P. H., Xu, J., Li, A., and Sun, Y. W.: Observation of CO2 Regional Distribution Using an Airborne Infrared Remote Sensing Spectrometer (Air-IRSS) in the North China Plain, Remote Sens.-Basel, 11, 123, 2019.
Wang, S. P., van der A, R. J., Stammes, P., Wang, W. H., Zhang, P., Lu, N. M., and Fang, L.: Carbon Dioxide Retrieval from TanSat Observations and Validation with TCCON Measurements, Remote Sens.-Basel, 12, 3626, https://doi.org/10.3390/rs12142204, 2020.
Wang, W., Tian, Y., Liu, C., Sun, Y., Liu, W., Xie, P., Liu, J., Xu, J., Morino, I., Velazco, V. A., Griffith, D. W. T., Notholt, J., and Warneke, T.: Investigating the performance of a greenhouse gas observatory in Hefei, China, Atmos. Meas. Tech., 10, 2627–2643, https://doi.org/10.5194/amt-10-2627-2017, 2017.
Wu, H., Wang, X. H., Ye, H. H., Jiang, Y., and Duan, F. H.: Error analysis of the greenhouse-gases monitor instrument short wave infrared XCO2 retrieval algorithm, J. Appl. Remote Sens., 12, 016015, https://doi.org/10.1117/1.JRS.12.016015, 2018.
Wunch, D., Toon, G. C., Wennberg, P. O., Wofsy, S. C., Stephens, B. B., Fischer, M. L., Uchino, O., Abshire, J. B., Bernath, P., Biraud, S. C., Blavier, J.-F. L., Boone, C., Bowman, K. P., Browell, E. V., Campos, T., Connor, B. J., Daube, B. C., Deutscher, N. M., Diao, M., Elkins, J. W., Gerbig, C., Gottlieb, E., Griffith, D. W. T., Hurst, D. F., Jiménez, R., Keppel-Aleks, G., Kort, E. A., Macatangay, R., Machida, T., Matsueda, H., Moore, F., Morino, I., Park, S., Robinson, J., Roehl, C. M., Sawa, Y., Sherlock, V., Sweeney, C., Tanaka, T., and Zondlo, M. A.: Calibration of the Total Carbon Column Observing Network using aircraft profile data, Atmos. Meas. Tech., 3, 1351–1362, https://doi.org/10.5194/amt-3-1351-2010, 2010.
Wunch, D., Toon, G. C., Blavier, J. F. L., Washenfelder, R. A., Notholt, J., Connor, B. J., Griffith, D. W. T., Sherlock, V., and Wennberg, P. O.: The Total Carbon Column Observing Network, Philos. T. Roy. Soc. A, 369, 2087–2112, 2011.
Wunch, D., Wennberg, P. O., Osterman, G., Fisher, B., Naylor, B., Roehl, C. M., O'Dell, C., Mandrake, L., Viatte, C., Kiel, M., Griffith, D. W. T., Deutscher, N. M., Velazco, V. A., Notholt, J., Warneke, T., Petri, C., De Maziere, M., Sha, M. K., Sussmann, R., Rettinger, M., Pollard, D., Robinson, J., Morino, I., Uchino, O., Hase, F., Blumenstock, T., Feist, D. G., Arnold, S. G., Strong, K., Mendonca, J., Kivi, R., Heikkinen, P., Iraci, L., Podolske, J., Hillyard, P. W., Kawakami, S., Dubey, M. K., Parker, H. A., Sepulveda, E., García, O. E., Te, Y., Jeseck, P., Gunson, M. R., Crisp, D., and Eldering, A.: Comparisons of the Orbiting Carbon Observatory-2 (OCO-2) measurements with TCCON, Atmos. Meas. Tech., 10, 2209–2238, https://doi.org/10.5194/amt-10-2209-2017, 2017.
Yang, D., Boesch, H., Liu, Y., Somkuti, P., Cai, Z., Chen, X., Di Noia, A., Lin, C., Lu, N., Lyu, D., Parker, R. J., Tian, L., Wang, M., Webb, A., Yao, L., Yin, Z., Zheng, Y., Deutscher, N. M., Griffith, D. W. T., Hase, F., Kivi, R., Morino, I., Notholt, J., Ohyama, H., Pollard, D. F., Shiomi, K., Sussmann, R., Te, Y., Velazco, V. A., Warneke, T., and Wunch, D.: Toward High Precision XCO2 Retrievals From TanSat Observations: Retrieval Improvement and Validation Against TCCON Measurements, J. Geophys. Res.-Atmos., 125, e2020JD032794, https://doi.org/10.1029/2020JD032794, 2020.
Yang, D. X., Liu, Y., Cai, Z. N., Chen, X., Yao, L., and Lu, D. R.: First Global Carbon Dioxide Maps Produced from TanSat Measurements, Adv. Atmos. Sci., 35, 621–623, 2018.
Yang, D. X., Liu, Y., Boesch, H., Yao, L., Di Noia, A., Cai, Z. N., Lu, N. M., Lyu, D. R., Wang, M. H., Wang, J., Yin, Z. S., and Zheng, Y. Q.: A New TanSat XCO2 Global Product towards Climate Studies, Adv. Atmos. Sci., 38, 8–11, 2021a.
Yang, D. X., Liu, Y., Feng, L., Wang, J., Yao, L., Cai, Z. N., Zhu, S. H., Lu, N. M., and Lyu, D. R.: The First Global Carbon Dioxide Flux Map Derived from TanSat Measurements, Adv. Atmos. Sci., 38, 1433–1443, 2021b.
Yang, Y., Zhou, M., Langerock, B., Sha, M. K., Hermans, C., Wang, T., Ji, D., Vigouroux, C., Kumps, N., Wang, G., De Mazière, M., and Wang, P.: New ground-based Fourier-transform near-infrared solar absorption measurements of XCO2, XCH4 and XCO at Xianghe, China, Earth Syst. Sci. Data, 12, 1679–1696, https://doi.org/10.5194/essd-12-1679-2020, 2020.
Yang, Z. D., Zhen, Y. Q., Yin, Z. S., Lin, C., Bi, Y. M., Liu, W., Wang, Q., Wang, L., Gu, S. Y., and Tian, L. F.: Laboratory spectral calibration of the TanSat atmospheric carbon dioxide grating spectrometer, Geosci. Instrum. Meth., 7, 245–252, 2018a.
Yang, Z. D., Zhen, Y. Q., Yin, Z. S., Lin, C., Bi, Y. M., Liu, W., Wang, Q., Wang, L., Gu, S. Y., and Tian, L. F.: Prelaunch Radiometric Calibration of the TanSat Atmospheric Carbon Dioxide Grating Spectrometer, IEEE T. Geosci. Remote, 56, 4225–4233, 2018b.
Yang, Z. D., Bi, Y. M., Wang, Q., Liu, C. B., Gu, S. Y., Zheng, Y. Q., Lin, C., Yin, Z. S., and Tian, L. F.: Inflight Performance of the TanSat Atmospheric Carbon Dioxide Grating Spectrometer, IEEE T. Geosci. Remote, 58, 4691–4703, 2020.
Yi, Y., Liu, Y., Cai, Z., Fang, S., Yang, D., Wang, Y., Liang, M., Yao, B., Ma, Q., and Wang, M.: Measuring and comparing in-situ CO2 and CO profiles with satellite observations and model data, Atmos. Ocean. Sc. Lett., 12, 444–450, https://doi.org/10.1080/16742834.2019.1649974, 2019.
Yoshida, Y., Kikuchi, N., Morino, I., Uchino, O., Oshchepkov, S., Bril, A., Saeki, T., Schutgens, N., Toon, G. C., Wunch, D., Roehl, C. M., Wennberg, P. O., Griffith, D. W. T., Deutscher, N. M., Warneke, T., Notholt, J., Robinson, J., Sherlock, V., Connor, B., Rettinger, M., Sussmann, R., Ahonen, P., Heikkinen, P., Kyrö, E., Mendonca, J., Strong, K., Hase, F., Dohe, S., and Yokota, T.: Improvement of the retrieval algorithm for GOSAT SWIR XCO2 and XCH4 and their validation using TCCON data, Atmos. Meas. Tech., 6, 1533–1547, https://doi.org/10.5194/amt-6-1533-2013, 2013.
Zellweger, C., Emmenegger, L., Firdaus, M., Hatakka, J., Heimann, M., Kozlova, E., Spain, T. G., Steinbacher, M., van der Schoot, M. V., and Buchmann, B.: Assessment of recent advances in measurement techniques for atmospheric carbon dioxide and methane observations, Atmos. Meas. Tech., 9, 4737–4757, https://doi.org/10.5194/amt-9-4737-2016, 2016.
Zhang, H., Zheng, Y. Q., Li, S., Lin, C., Li, C. L., Yuan, J. Z., and Li, Y.: Geometric correction for TanSat atmospheric carbon dioxide grating spectrometer, Sensor Actuat. A-Phys., 293, 62–69, 2019.
Zhang, X. Y., Wang, F., Wang, W. H., Huang, F. X., Chen, B. L., Gao, L., Wang, S. P., Yan, H. H., Ye, H. H., Si, F. Q., Hong, J., Li, X. Y., Cao, Q., Che, H. Z., and Li, Z. Q.: The development and application of satellite remote sensing for atmospheric compositions in China, Atmos. Res., 245, 105056, https://doi.org/10.1016/j.atmosres.2020.105056, 2020.
Zhang, Y. Z., Gautam, R., Pandey, S., Omara, M., Maasakkers, J. D., Sadavarte, P., Lyon, D., Nesser, H., Sulprizio, M. P., Varon, D. J., Zhang, R. X., Houweling, S., Zavala-Araiza, D., Alvarez, R. A., Lorente, A., Hamburg, S. P., Aben, I., and Jacob, D. J.: Quantifying methane emissions from the largest oil-producing basin in the United States from space, Science Advance, 6, eaaz5120, https://doi.org/10.1126/sciadv.aaz5120, 2020.
Zhao, M. J., Si, F. Q., Zhou, H. J., Jiang, Y., Ji, C. Y., Wang, S. M., Zhan, K., and Liu, W. Q.: Pre-Launch Radiometric Characterization of EMI-2 on the GaoFen-5 Series of Satellites, Remote Sens.-Basel, 13, 2843, 2021.
Zheng, B., Chevallier, F., Ciais, P., Broquet, G., Wang, Y., Lian, J., and Zhao, Y.: Observing carbon dioxide emissions over China's cities and industrial areas with the Orbiting Carbon Observatory-2, Atmos. Chem. Phys., 20, 8501–8510, https://doi.org/10.5194/acp-20-8501-2020, 2020a.
Zheng, B., Geng, G. N., Ciais, P., Davis, S. J., Martin, R. V., Meng, J., Wu, N. N., Chevallier, F., Broquet, G., Boersma, F., Van Der A, R., Lin, J. T., Guan, D. B., Lei, Y., He, K. B., and Zhang, Q.: Satellite-based estimates of decline and rebound in China's CO2 emissions during COVID-19 pandemic, Science Advances, 6, eabd4998, https://doi.org/10.1126/sciadv.abd4998, 2020b.
- Abstract
- Introduction
- History of GHG monitoring around the globe
- Status of GHG monitoring in China
- Advances in GHG monitoring in China
- Challenges
- Future perspectives
- Conclusions
- Data availability
- Author contributions
- Competing interests
- Disclaimer
- Acknowledgements
- Financial support
- Review statement
- References
- Supplement
- Abstract
- Introduction
- History of GHG monitoring around the globe
- Status of GHG monitoring in China
- Advances in GHG monitoring in China
- Challenges
- Future perspectives
- Conclusions
- Data availability
- Author contributions
- Competing interests
- Disclaimer
- Acknowledgements
- Financial support
- Review statement
- References
- Supplement