the Creative Commons Attribution 4.0 License.
the Creative Commons Attribution 4.0 License.
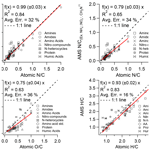
Enhancing characterization of organic nitrogen components in aerosols and droplets using high-resolution aerosol mass spectrometry
Xinlei Ge
Justin Trousdell
Mindong Chen
This study aims to enhance the understanding and application of the Aerodyne high-resolution aerosol mass spectrometer (HR-AMS) for the comprehensive characterization of organic nitrogen (ON) compounds in aerosol particles and atmospheric droplets. To achieve this goal, we analyzed 75 N-containing organic compounds, representing a diverse range of ambient non-organonitrate ON (NOON) types, including amines, amides, amino acids, N heterocycles, protein, and humic acids. Our results show that NOON compounds can produce significant levels of and ion fragments, which are typically recognized as ions representative of inorganic nitrogen species. We also identified the presence of CH2N+ at = 28.0187, an ion fragment rarely quantified in ambient datasets due to substantial interference from . As a result, the utilization of an updated calibration factor of 0.79 is necessary for accurate NOON quantification via the HR-AMS. We also assessed the relative ionization efficiencies (RIEs) for various NOON species and found that the average RIE for NOON compounds (1.52 ± 0.58) aligns with the commonly used default value of 1.40 for organic aerosol. Moreover, through a careful examination of the HR-AMS mass spectral features of various NOON types, we propose fingerprint ion series that can aid the NOON speciation analysis. For instance, the presence of ions is closely linked with amines, with CH4N+ indicating primary amines, C2H6N+ suggesting secondary amines, and C3H8N+ representing tertiary amines. CnH2nNO+ ions (especially for n values of 1–4) are very likely derived from amides. The co-existence of three ions, , C2H3NO+, and CH4NO+, serves as an indicator for the presence of amino acids. Additionally, the presence of ions indicates the occurrence of 2N-heterocyclic compounds. Notably, an elevated abundance of is a distinct signature for amines and amino acids, as inorganic ammonium salts produce only negligible amounts of in the HR-AMS.
Finally, we quantified the NOON contents in submicron particles (PM1) and fog water in Fresno, California, and PM1 in New York City (NYC). Our results revealed the substantial presence of amino compounds in both Fresno and NYC aerosols, whereas concurrently collected fog water in Fresno contained a broader range of NOON species, including N-containing aromatic heterocycle (e.g., imidazoles) and amides. These findings highlight the significant potential of employing the widespread HR-AMS measurements of ambient aerosols and droplets to enhance our understanding of the sources, transformation processes, and environmental impacts associated with NOON compounds in the atmosphere.
- Article
(2882 KB) - Full-text XML
-
Supplement
(881 KB) - BibTeX
- EndNote
Nitrogen-containing organic species (ON) have been ubiquitously observed in atmospheric particles and aqueous phases (e.g., fog/cloud droplets and rainwater). These compounds can constitute a significant portion (∼ 30 %) of the total airborne nitrogen (Cape et al., 2011) and contribute to up to 25 % of the total nitrogen (N) deposition flux (Jickells et al., 2013; Kanakidou et al., 2016). However, our understanding of atmospheric ON is generally limited compared to inorganic N species such as ammonium, nitrate, and nitrite. This disparity arises from the diverse nature of ON, which encompasses a wide range of compounds with varying carbon numbers, functional groups, and physicochemical properties. ON compounds, originating from multiple emission sources and formed through diverse chemical processes, exhibit notable spatial and temporal variations in the atmosphere. The presence of airborne ON carries significant implications for regional air quality, earth's climate, the terrestrial and aquatic ecosystem, and human health (Cape et al., 2011; Cornell et al., 1995; Liu et al., 2017). Among these compounds, aliphatic amines have been found to play a significant role in new particle formation and growth (Smith et al., 2010; Almeida et al., 2013; Yao et al., 2018) and contribute to secondary organic aerosol formation (Murphy et al., 2007; Yu et al., 2017; Song et al., 2017). Furthermore, ON species have been demonstrated to significantly influence the optical and hygroscopic properties of aerosols (Powelson et al., 2014; Lee et al., 2013; Rovelli et al., 2017), thereby influencing cloud formation and the atmospheric water cycle. These observations highlight the importance of studying ON compounds and their behaviors in the atmosphere.
Bulk ON, which represents the nitrogen content within organic compounds, is typically quantified by subtracting the inorganic nitrogen (IN) content from that of the total nitrogen (TN): ON = TN − IN. IN is the combined nitrogen amount in ammonium, nitrate, and nitrite (Zhang and Anastasio, 2001). Measurement of TN involves converting N-containing species into inorganic ions (e.g., , , and ) or nitrogen gases (e.g., NOx and NH3) using chemical, photochemical, or high-temperature oxidation approaches (Cornell et al., 2003; Zhang and Anastasio, 2003a; Jickells et al., 2013). However, quantifying ON using differing methods can introduce significant uncertainties, due to factors such as incomplete transformation of ON compounds and aggregation of measurement errors (Cornell et al., 2003; Cape et al., 2011). The latter is especially important when ON is minor compared to IN in the sample. Moreover, ON measurements have traditionally focused on the water-soluble fraction (WSON). However there are observations that water-insoluble ON (WION) can constitute a large fraction of the total ON and, sometimes, may be more important than WSON (Miyazaki et al., 2011; Russell et al., 2003). Furthermore, most studies investigating atmospheric ON have relied on samples collected over periods of hours to days. The limited time resolution of these measurements hampers the ability to capture the rapid evolution processes of ON species in the atmosphere.
The speciation of atmospheric ON has been conducted using various techniques, including ion chromatography (Vandenboer et al., 2011; Verriele et al., 2012; Parworth et al., 2017; Liu et al., 2021; Place et al., 2017), gas chromatography coupled with different detectors (Özel et al., 2011, 2009; Akyüz, 2007), infrared (IR) and nuclear magnetic resonance (NMR) spectroscopy (Herckes et al., 2007), liquid chromatography with mass spectrometry (LC-MS) (Ruiz-Jimenez et al., 2012; Samy and Hays, 2013; Samy et al., 2011; Ye et al., 2017), high-resolution electrospray ionization mass spectrometry (ESI-MS) (Laskin et al., 2009; Altieri et al., 2012; Rincon et al., 2012; Shi et al., 2020), aerosol mass spectrometry (Junninen et al., 2010; Huang et al., 2012; Setyan et al., 2014; Zhou et al., 2016; Ge et al., 2014; Xu et al., 2017; Huang et al., 2021; Yu et al., 2019), chemical ionization mass spectrometry (Yao et al., 2018; Zheng et al., 2015; Yao et al., 2016; Yu and Lee, 2012), and nano-secondary ion mass spectrometry (Li et al., 2016). It is important to note that no single technique can comprehensively capture the full spectrum of ON species. However, certain mass spectrometric techniques may provide broader coverage (Cape et al., 2011). A variety of ON species have been detected in the atmosphere, including amines, amino acids, urea, amides, nitriles, organonitrates, nitro-compounds, and N-heterocyclic compounds. However, when considered individually, these identified ON classes often contribute only a small fraction to the overall ON pool. For example, amino compounds, including amines, amino acids, peptides, and proteins, were found to constitute less than 20 % of the total ON in the Central Valley of California – a region known for intense agricultural emissions of these compounds (Zhang and Anastasio, 2001; Zhang et al., 2002). Moreover, in some instances, ON compounds were only qualitatively characterized without quantitative information. These limitations restrict a comprehensive understanding of the chemistry of atmospheric ON compounds and their environmental impacts.
The Aerodyne high-resolution time-of-flight aerosol mass spectrometer (HR-AMS) is a highly promising tool for characterizing ON in atmospheric condensed phases. It utilizes thermal vaporization and electron impact (EI) ionization techniques to quantify aerosol components and has been widely used for real-time measurements of non-refractory components in submicron aerosols (PM1) with fast time resolution (Zhang et al., 2020; Decarlo et al., 2006). An important feature of the HR-AMS is its ability to differentiate fragment ions with the same integer mass-to-charge ratio () but slightly different exact masses. This capability allows for the determination of elemental compositions of the detected ions (Decarlo et al., 2006) and the average atomic ratios, such as oxygen-to-carbon (), hydrogen-to-carbon (), and nitrogen-to-carbon (), for the bulk organic aerosol (OA) (Aiken et al., 2008; Ma et al., 2021). These ratios are particularly valuable for estimating the total ON content present in aerosol samples.
While the speciation analysis of ON using the HR-AMS mass spectra (MS) can be challenging due to the extensive fragmentation of parent molecules (Drewnick, 2012), it is still possible to identify different types of ON by leveraging unique spectral fingerprints, such as those observed for aliphatic amines (Murphy et al., 2007; Sun et al., 2011), N heterocycles (Hawkins et al., 2018; Kim et al., 2019), and organonitrates (Farmer et al., 2010). Furthermore, the HR-AMS offers a new perspective for source identification of ON in ambient air. Specifically, the highly time-resolved HR-AMS data, typically collected at intervals of 2–5 min during field studies, offer valuable insights into the co-variation of ON species with other known components and permit the application of multivariate factor analysis techniques to differentiate components that exhibit similar patterns of behavior. A recent study has successfully applied positive matrix factorization (PMF) on the N-containing ions in an HR-AMS field dataset and determined ON factors that can facilitate the inference of specific sourced or formation processes (Qi et al., 2022).
To achieve a comprehensive characterization of atmospheric ON using the HR-AMS, it is necessary to optimize and validate the technique. While the interpretation of OA behaviors has often relied on the and ratios (Aiken et al., 2008; Canagaratna et al., 2015), the ratio has received limited attention, and previous AMS measurements on ON standards are scarce. The work by Aiken et al. (2008) includes 27 ON compounds; however, ON spectra were not reported. These spectra are reported in this study. Ge et al. (2014) introduced a method using the HR-AMS to characterize amines and their degradation products in postcombustion CO2 capture (PCCC) processes. In that study, we performed analysis of the AMS spectra of 12 amino compounds and the NIST spectra for 37 ON compounds that were identified as the degradation products from PCCC amines. In a separate study, Price et al. (2023) tested the relative ionization efficiencies (RIEs) of three ON compounds. Additionally, Farmer et al. (2010) and Day et al. (2022) proposed AMS-based methods for quantifying organonitrates (a.k.a. organic nitrates), which have been broadly adopted by various AMS studies (e.g., Huang et al., 2021; Yu et al., 2019; Xian et al., 2023; Lin et al., 2021; Zhu et al., 2016).
To address the knowledge gap, this study conducted analyses on a large set of ON standards, encompassing diverse chemical types likely present in the atmosphere. The HR-AMS spectra obtained were meticulously analyzed to characterize N-containing ion fragments and establish the relationship between the ratios measured by the HR-AMS and the nominal values of the compounds. In addition, we carefully investigated mass spectral features of the ON standards and proposed speciation analysis protocols. Furthermore, we evaluated the effectiveness and applicability of the proposed method by examining three ambient HR-AMS datasets. Our results demonstrate that the optimized HR-AMS technique and the proposed analysis protocols carry great promise at enhancing our ability to investigate the sources, transformation processes, and environmental impacts of atmospheric ON.
A comprehensive set of 75 ON standards were analyzed by the HR-AMS, and detailed information regarding these standards can be found in Table S1 in the Supplement. These standards, which include 27 amines (no. 1–27), 6 amides (no. 28–33), 27 amino acids (no. 34–59 and 71), 4 nitro-compounds (no. 60–63), 7 N-containing heterocycles (no. 64–70), 1 protein (no. 72), and 3 humic-like substances (no. 73–75), were mostly procured from Sigma-Aldrich with purities exceeding 98 %. Note that organonitrates are not included because the fragmentation of organonitrates in the AMS predominantly generates ions (generally > 50 %, mostly NO+ at 30) and organic moieties that lack nitrogen. These ions cannot be used as fingerprint ions for quantifying organonitrates. Instead, the quantification of organonitrates is commonly achieved based on the differences of ratios between organic nitrates and ammonium nitrate (Farmer et al., 2010; Day et al., 2022). For clarity, we denote the compounds studied here as non-organonitrate ON (NOON). In addition, to probe the RIEs of different NOON species, we analyzed 18 mixtures containing sulfate (in the form of ammonium sulfate) (Fisher Chemicals, > 99 %) and individual NOON standards in a 1:1 mass ratio (Table S2). All solutions were prepared using purified water (> 18.2 MΩ cm) obtained from a Milli-Q system (Millipore, USA).
The procedures for HR-AMS analysis of liquid samples have been comprehensively documented in prior works (Sun et al., 2010; Ge et al., 2014, 2017; O'Brien et al., 2019; Niedek et al., 2023). Each NOON standard was carefully weighted, dissolved in Milli-Q water, and diluted to a solute concentration of ∼ 20 ppm. A portion of the solution (20–40 mL) was dispensed into a sample vial and then nebulized using a collision-type atomizer, with high-purity argon as the carrier gas. Aerosol particles were dehydrated in a diffusion dryer filled with silica-gel, reducing the relative humidity (RH) to < 5 %, before introduction into the HR-AMS. The HR-AMS was operated at a vaporizer temperature of ∼ 600 ∘C and was alternated between the highly sensitive V mode and the high-mass-resolution W mode ( ∼ 5000). For this study, W-mode data with an extended range up to 500 amu were used, aligning with our focus on characterizing the chemical composition. To eliminate the interference of the signal on CH2N+ at = 28.033 and CO+ at 28.01, the aerosol generation system was initially purged by atomizing Milli-Q water under argon until the signal measured by the HR-AMS reached a sufficiently low level. HR-AMS data for each sample were recorded under stable particle flow conditions, and the high-resolution mass spectrum (HRMS) of each NOON standard was derived from the average of at least three stable runs, each lasting 150 s. To account for any potential contamination or background signals, a blank measurement was conducted by aerosolizing Milli-Q water between every two samples, following the same procedure. Typically, the measured signals of Milli-Q water were minimal and had negligible influence on the samples.
The HR-AMS data were analyzed using the standard AMS data analysis software, namely SQUIRREL v1.46/v1.51H and PIKA v1.06/v1.10H (https://cires1.colorado.edu/jimenez-group/ToFAMSResources/ToFSoftware/index.html (last access: 11 January 2024), written in Igor Pro (Wavemetrics, Portland, USA). During data post-processing, measures were taken to eliminate interference caused by air components on organic fragments (e.g., at 28, at 32). Given that the RH values recorded during the measurements remained consistently very low (< 5 %), the influence of particle-bound water was deemed negligible. Thus, the mass spectral analyses were conducted using the directly measured signals of O+, OH+, H2O+, and CO+.
HR-AMS data acquired from the online measurements of PM1 during July–August 2009 in NYC (Sun et al., 2011) and during January 2010 in Fresno, CA (Ge et al., 2012b, a), were used in this study. Additionally, we examined a set of 11 fog water samples collected during 9–16 January 2010, in Fresno. These fog waters were collected using two Caltech Active Strand Cloudwater Collector (CASCC) instruments. Immediately after collection, the fog water samples were filtrated with 0.45 µm syringe filters (Pall Laboratory) and then stored in pre-cleaned high-density polyethylene (HDPE) bottles within a freezer at −20 ∘C until analysis. The analysis of fog water samples involved the utilization of the same HR-AMS instrument and a similar procedure employed for the NOON standards. More details on fog water analysis can be found in Kim et al. (2019). The analytical technique developed in this work was applied on these datasets to assess its effectiveness and applicability.
3.1 N C calibration factor
Accurate determination of elemental ratios using HR-AMS spectra requires calibrations due to the loss of neutral fragments and the inability to detect very small ions such as H+. The calibration factor for reported in Aiken et al. (2008) was established based on 27 ON standards, mainly consisting of amino acids; the ratios derived from HR-AMS spectra for these standards agree well with the nominal values (slope = 0.96; r2 = 0.95), and the estimated uncertainty associated with this calibration was 22 %. In this study, we re-evaluated the calibration factor using a considerably larger dataset comprising 75 standards that represent a broad range of N-containing functional groups (see Table S1). The new calibration plot (Fig. 1a) shows a similar slope (0.99 ± 0.03, r2 = 0.84), albeit with a higher uncertainty of 32 % compared to the previous calibration. This increased uncertainty is partially attributed to the large positive biases observed for a few primary amines (further details provided in Sect. 3.4). In addition, the calibration factors for (0.75) and (0.93) (Fig. 1c and d), determined based on the NOON standards in this study, exhibit good agreements with those reported by Aiken et al. (2008) (0.75 for and 0.91 for ).
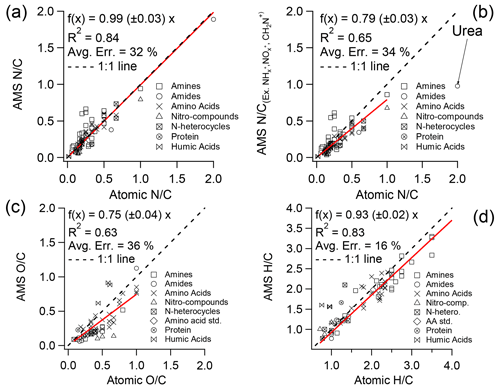
Figure 1Atomic ratios from the elemental analysis of 75 NOON standards versus the nominal values by (a) including and (b) excluding ion categories of , , and CH2N+ and the atomic ratios of (c) and (d) from the same set of standards versus nominal values. Table S1 provides details of all the standards used here. Note that urea is not included in the linear regression in (b), and the linear regressions are orthogonal distance regression constrained to pass through the origin. The “average error” (Avg. Err.) represents the mean of the absolute relative deviations of all samples from the fitted red line in the corresponding figure.
Table 1Average values with ± 1 standard deviation for the percent mass contributions of major N-containing ion categories to the total mass of various types of organic nitrogen standards. Note that M+ refers to the molecular ion of the compound; for example, M+ represents CH5N+ for methylamine. Numbers in bold emphasize relatively large and significant values.
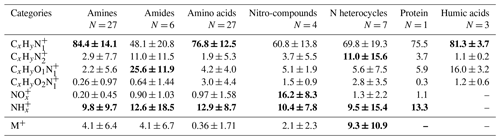
However, it should be noted that the calibration factors reported in Fig. 1a (0.99) and in Aiken et al. (2008) (0.96) include contributions from and ions. Yet, these ions are typically assigned to inorganic ammonium and nitrate, respectively, during the analysis of ambient AMS data. Consequently, adjustments should be made to the correction factors to ensure accurate estimation of NOON content in ambient aerosols. Table 1 shows that ions are consistently observed in the MS of various types of NOON standards, contributing approximately 10 % to the total N content on average. ions are particularly significant in nitro-compounds, contributing on average 16.2 (± 8.3)% to the total N. Based on the standards analyzed in this study, excluding the contributions from and would lead to an overall reduction of ∼ 13 % in the calibration ratio. In addition, the CH2N+ ion ( = 28.0187) is an important fragment in most NOON compounds, particularly amines. However, due to interference from the adjacent ion, it is necessary to exclude the CH2N+ ion in the analysis of ambient HR-AMS data. By excluding this ion, the calibration ratio would further decrease by ∼ 7 %.
Considering the exclusion of , , and CH2N+ ions, we have derived a new calibration factor of 0.79 (Fig. 1b). We highly recommend using this value to determine the ratio for ambient samples. It should be noted again that this new ratio is for NOON compounds; there may be underestimations of the ON content in cases where aerosols/droplets contain a significant amount of organonitrates. The underestimation also occurs in case of a significant presence of urea because a large fraction of the N mass (generally > 50 %) is present in for urea.
3.2 Relative ionization efficiencies (RIEs) of different NOON species
For the AMS measurements, relative ionization efficiency (RIE) is defined as the ratio of the ionization efficiency of a species to that of nitrate, quantified in terms of mass (Canagaratna et al., 2007); it is crucial for accurately quantifying the mass of the specific species in question. Here, the RIEs of NOON species were determined by analyzing the HR-AMS spectra of 18 mixtures containing NOON species and sulfate in a 1:1 mass ratio. The measured ratios between the total organic signal and the sulfate signal varied from approximately 0.3 to 2, with an average of 1.33 (Fig. S1 in the Supplement). Assuming that the aerosol composition measured by the HR-AMS corresponds to the solute composition in the original mixtures, these ratios represent the RIEs of different NOON compounds relative to sulfate. In this study, since the RIE of sulfate relative to nitrate (based on mainly NO+ and ) was found to be 1.14, the average RIE of NOON species relative to nitrate was determined to be 1.52 ± 0.58. Varying mass ratios of NOON to sulfate in the solutions should have negligible influence on the determined RIE value. It is important to note that the obtained result from the analysis of 18 mixtures aligns very well with the average RIE of 1.6 ± 0.5 reported by Price et al. (2023), which was based on a study involving three compounds (4-nitrocatechol, isosorbide mononitrate, and triammonium citrate). This RIE value (1.52) is only ∼ 8.6 % higher than the widely used default value of 1.4 for the quantification of total OA (Canagaratna et al., 2007). However, considering the inherent measurement uncertainties, such as variations in volatility, leading to deviations in HR-AMS-determined particle compositions from the original mixture ratios, and the potential influences of impurities in the solutions on the measured mass of NOON and thus the resulting RIE, we recommend maintaining the default RIE of 1.4 for the quantification of NOON in ambient aerosols and droplets.
3.3 Quantification of NOON in ambient aerosols/droplets
The mass concentration of NOON can be determined based on HR-AMS measurements using the following equations:
where OC represents the mass concentration of carbon present in OA, OMmass denotes the measured total OA mass, and ONNOON denotes the mass of nitrogen in NOON species. The (organic mass to organic carbon) and ratios (calculated using the new correction factor of 0.79) can be derived from HR-AMS elemental analysis.
For liquid samples, such as aqueous extracts of collected filter samples, fog, cloud, and rainwater, the ONNOON concentrations can be determined (in ppm or mg L−1) using the OC content measured by a total organic carbon (TOC) analyzer, typically representing the water-soluble OC (WSOC) (in ppm or mg L−1). The corresponding WSONNOON concentration can be calculated as follows:
where the factor () is the ratio of the molar masses of nitrogen and carbon.
In the above calculations, accurate quantification of ONNOON relies on the accuracy of determination, which can be influenced by the need to estimate HxO+ (mainly H2O+, OH+, O+) and CO+ signals for ambient datasets (Aiken et al., 2008). The HxO+ signals do not directly affect the ratio and hence have no impact on the WSONNOON calculation in Eq. (3). However, the current estimation of HxO+ (H2O+ = 0.225 , OH+ = 0.25 H2O+, O+ = 0.05 H2O+) for ambient OA (Aiken et al., 2008) can introduce uncertainties in the and ratios, thereby influencing the accuracy of the ratio. If Eq. (1) is used to calculate the OC content, an overestimation of the ratio would lead to a lower OC and an underestimation of ONNOON in Eq. (2). The estimation of CO+ (i.e., ; Aiken et al., 2008) can also affect the elemental ratios and ONNOON quantification, albeit to a lesser extent compared to the HxO+ ions. These uncertainties can be minimized, though not entirely eliminated, by conducting measurements on HEPA-filtered ambient air during field campaigns.
Additionally, in the HR-AMS spectra of ambient samples, ON ions are typically observed at substantially lower intensities compared to adjacent ions and are often located at the edges or between and ions. For instance, ions are often found between and ions, while ions are located between and ions (refer to Fig. S2 for two examples at 42 and 44). Therefore, accurate determination of ON ions requires careful optimization of the peak shape and peak width parameters prior to performing peak fittings. This optimization helps reduce uncertainties associated with fitting of ON ions, particularly those with low signal intensities. Furthermore, caution should be given when fitting ON ions with high values (e.g., > 100 amu) since the mass resolution of the HR-AMS may not be sufficient to unambiguously distinguish ON ions from neighboring high isobaric ions.
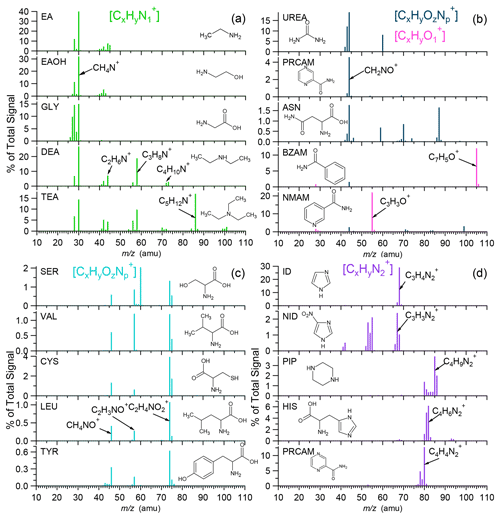
Figure 2Characteristic spectral peaks of selected NOON standards with different functional groups of (a) amines (R–NH2, R2–NH, R3–N), (b) amides (R–CO–NH2), (c) amino acids (R–CH(NH2)–COOH), and (d) 2N heterocycles (note that the full HRMS of these compounds can be found in Fig. S3). EA: ethylamine. EAOH: ethanolamine. GLY: glycine. DEA: diethylamine. TEA: triethylamine. UREA: urea. PRCAM: pyrazinecarboxamide. ASN: asparagine. BZAM: benzamide. NMAM: N,N'-methylenebisacrylamide. SER: serine. VAL: valnine. CYS: cysteine. LEU: leucine. TYR: tyrosine. ID: imidazole. NID: nitro-imidazole. PIP: piperazine. HIS: histidine.
3.4 HR-AMS mass spectral features of NOON compounds
Detailed analyses of the HR-AMS spectra of all tested NOON standards (Fig. S3) reveal unique fragmentation patterns associated with specific functional groups. Aliphatic amines, characterized by the strong electron-donating ability of the nitrogen atom, predominantly undergo α cleavage, resulting in the formation of ion series of , e.g., CH4N+ ( 30.0344), C2H6N+ ( 44.0500), C3H8N+ ( 58.0657), C4H10N+ ( 72.0813), and C5H12N+ ( 86.0970), depending on the molecular structures (Mclafferty and Turecek, 1993). Notably, primary amino compounds with the –CH2–NH2 functional group, such as ethylamine, ethanolamine, and glycine (Fig. 2a), exhibit the most abundant peak of CH4N+ ( 30.0344). In the case of butylamine, iso-butylamine, and pentylamine, the CH4N+ ion can constitute > 60 % of the total signals in their respective MS, leading to significant positive biases in the ratios determined by the HR-AMS, as shown in Fig. 1a and b. Additionally, secondary elimination from initial α cleavage may contribute significantly to the formation of ions for secondary and tertiary amines (e.g., diethylamine and triethylamine; Fig. 2a).
α cleavage can be an important fragmentation pathway for amides as well, causing the loss of alkyl groups and the formation of ion series of CnH2nNO+, such as CH2NO+ ( 44.0136), C2H4NO+ ( 58.0293), C3H6NO+ ( 72.0449), and C4H8NO+ ( 86.0606) (Mclafferty and Turecek, 1993). Particularly, the CH2NO+ ion (sometimes also CHNO+) can be generated from the amide group (-CO-NH2), as observed for urea, pyrazinecarboxamide, and L-asparagine (Fig. 2b). Additionally, cleavage of the C(O)–N bond can be significant for certain amides, resulting in the formation of C3H3O+ from acetaminophenol and C7H5O+ from benzamide (Fig. 2b).
Amino acids containing the functional group –CH(NH2)–COOH commonly produce significant signals of ( 74.0242) resulting from α cleavage. Subsequent losses of –OH and –CO from the ion lead to the formation of C2H3NO+ ( 57.0215) and CH4NO+ ( 46.0293), respectively. This pattern is observed in amino acids such as serine, valine, cysteine, leucine, and tyrosine, as depicted in Fig. 2c.
2N-Heterocyclic compounds show characteristic peaks in their spectra (Fig. 2d). For example, imidazole and nitro-imidazole produce ions at 67.0296/68.0374 (), while histidine generates at 81.0452/82.0531. The imidazolyl compounds also produce ion series of (n > 1). On the other hand, compounds containing the pyrazinyl group show notable series, with at 80.0374 representing pyrazinecarboxamide.
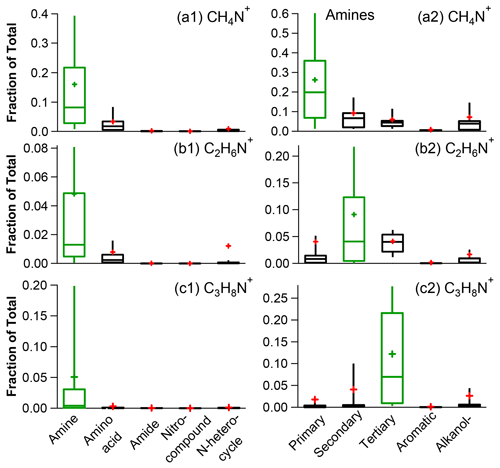
Figure 3Fractional contributions of CH4N+ (a), C2H6N+ (b), and C3H8N+ (c) to the mass spectra of the N-containing organic species with different functional groups (left panel, a1, b1 and c1) and different classes of amines (right panel, a2, b2 and c2) (the whiskers above and below the boxes indicate the 90th and 10th percentiles, the upper and lower boundaries of the boxes indicate the 75th and 25th percentiles, the lines in the boxes indicate the median values, and the cross symbols indicate the mean values).
Nitro-compounds containing the –NO2 group (e.g., nitrophenol, 2-nitrobenzaldehyde and 2-nitrobenzyl alcohol) display a much higher ratio (averaging 8.64 for the four compounds tested in this study). This ratio is similar to that observed for organic nitrates (i.e., RONO2) (Farmer et al., 2010). In comparison, the ratio in pure ammonium nitrate is much lower, which is 2.69 in this work, ∼ 2.7 in Fry et al. (2009), and 2.4 in Bruns et al. (2010). In addition, although not measured in this study, nitriles have been shown to generate principal ion series of and (Mclafferty and Turecek, 1993).
In the HR-AMS spectra of NOON compounds, the contributions of molecular ions are generally low, averaging around 3.3 % of the total signals (see average contributions of different NOON categories in Table 1). The relationship between the compounds' molecular weights and the abundance of molecular ions is not straightforward (Fig. S4 in the Supplement). However, for compounds with stable structures including benzene or heterocyclic rings, a high abundance of molecular ion peak is typically observed. For example, pyrazole and imidazole exhibit a prominent peak at 68.0374 (21.2 % for pyrazole and 28.2 % for imidazole), 1,3-phenylenediamine shows a peak at 108.0687 (20.6 %), 4-aminophenol displays a C6H7NO+ peak at 109.0528 (17.1 %), and nicotinamide exhibits a C6H6N2O+ peak at 122.0480 (5.8 %). It is worth noting that a few simple and low-molecular-weight aliphatic amines, such as methylamine (17.0 %), dimethylamine (15.8 %), and trimethylamine (12.4 %), and urea (a simple amide) (8.1 %) also show relatively high contributions from molecular ions in their HR-AMS spectra as well.
3.5 Speciation analysis of NOON in ambient aerosols and droplets
The analysis of NOON compounds or classes in AMS spectra of ambient samples presents challenges due to their low concentrations and the complexities and uncertainties involved in assigning ion fragments to potential parent molecules. However, by examining the spectral features as discussed earlier, we can effectively address these challenges. To provide a comprehensive overview, we have compiled the mass contributions of different N ion categories to the total ON mass for different types of NOON compounds and summarized them in Table 1.
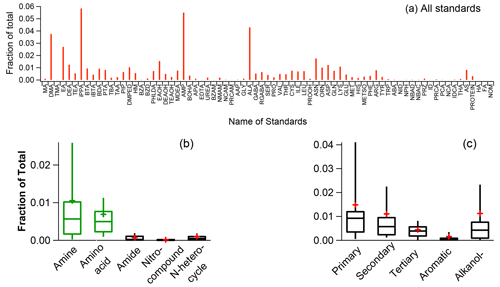
Figure 4Mass fractional contributions of in the HR-AMS spectra for all standards (a), different NOON classes (b), and different amines (c). In panels (b) and (c), whiskers above and below the boxes indicate the 90th and 10th percentiles, the upper and lower boundaries of the boxes indicate the 75th and 25th percentiles, the lines in the boxes indicate the median values, and the cross symbols indicate the mean values.
With the exception of amides, the majority of ON mass originates from ions, accounting for over 60 % of the total. This is particularly true for amines and amino acids. Considering the atmospheric abundances of amines (Ge et al., 2011a, b), the significant presence of ions strongly suggests the presence of amines. Moreover, ions may indicate the existence of aliphatic amines. To evaluate this further, we calculated the fractional contributions of three specific ions – CH4N+, C2H6N+, and C3H8N+ (left panel of Fig. 3) – in the HR-AMS MS of different NOON classes. It is evident that these three ions are clearly abundant in amines rather than in other types of N-containing species. Furthermore, a close examination of the distribution of these ions among different types of amines (right panel of Fig. 3) reveals that CH4N+ is mainly associated with primary amines (e.g., methylamine, ethylamine, butylamine, pentylamine, and ethanolamine), C2H6N+ is more likely linked with secondary amines (e.g., dimethylamine and diethylamine), and C3H8N+ is mainly produced by tertiary amines (e.g., trimethylamine and triethylamine).
Another intriguing observation is that only amines and amino acids can generate significant ( 18.0344) signal compared to other NOON standards (Fig. 4). Conversely, the HR-AMS spectra of pure ammonium nitrate, ammonium sulfate, and ammonium chloride (Fig. S6) do not produce the ion. This disparity is likely due to the cleavage of the C–N bond, accompanied by hydrogen migration to the () fragments. Importantly, this finding strongly indicates that can serve as an HR-AMS marker for the identification of amino compounds, including both amines and amino acids.
In addition, the contribution of ions to the ON mass is generally small (< 6 %), except for amides, where it can reach ∼ 26 % (Table 1). This observation suggests that the abundance of ions, particularly CH2NO+, can serve as an indicator of the presence of amides. In addition, we propose that the co-occurring ( 74.0242), C2H3NO+ ( 57.0215), and CH4NO+ ( 46.0293) can act as tracer ions for amino acids. Furthermore, an enrichment of ions suggests the presence of certain 2N-heterocyclic compounds, such as imidazoles and pyrazines (Kim et al., 2019; Hawkins et al., 2018). Finally, a high ratio is likely a signature indicating the abundance of nitro-compounds/organic nitrates (Farmer et al., 2010; Fry et al., 2009).
Since the AMS uses standard 70 eV electron ionization (EI), the obtained spectra are expected to be comparable with the EI spectra available in the NIST database (National Institute of Standards and Technology Standard Reference Database 1A, NIST 11, Software version 2.0g). Figure S5 shows the correlation coefficients (Pearson's r2) between the AMS-measured spectra (summed in unit mass resolution) and the NIST spectra for the 75 NOON standards. The level of spectral similarity varies among different types of NOON compounds. Higher correlations are observed for amines (average r2 = 0.70) and amides (average r2 = 0.63), while amino acids exhibit low correlations (average r2 = 0.23). The r2 values for N heterocycles and nitro-compounds are 0.57 and 0.50, respectively. Although an increase in molecular weight generally tends to decrease the spectral similarities, the influence of molecular weight is not particularly evident (Fig. S5).
A more detailed analysis reveals that the AMS spectra of aromatic compounds with ring structures exhibit close agreements with the corresponding NIST spectra (e.g., 1,3-phenylenediamine, 4-aminophenol, and 2-picolinic acid in Fig. S7). Conversely, spectra of aliphatic molecules (long-chain or branched) show less similarity and contain more signals towards the low range compared to the NIST spectra (e.g., tri-n-amylamine and lysine in Fig. S7). Additionally, AMS spectra of oxygenated compounds tend to deviate more significantly from their NIST counterparts than other compound types. This divergence can be attributed to additional thermal energy provided by the 600 ∘C AMS oven, resulting in more extensive fragmentation compared to the NIST spectra. Indeed, previous investigations by our group have demonstrated that employing a lower AMS vaporizer temperature (Tvap) (e.g., 250 ∘C) can reduce fragmentation, enhance the signals of parent molecular ions and/or fingerprint fragments, and overall increase the resemblance to the corresponding NIST spectra (Ge et al., 2014). Docherty et al. (2015) also showed that the OA MS changes substantially with variations in Tvap, and utilizing a lower Tvap can improve the analysis of some reduced OA species (with more ions). Therefore, during field deployments (or offline laboratory studies), it would be valuable to periodically acquire AMS spectra at lower Tvap in addition to the standard 600 ∘C spectra, as they may provide richer chemical information for compound speciation.
3.6 Characterization of NOON in ambient aerosols and fog water
Using the new calibration factor (0.79), we determined the ratios of NYC PM1, Fresno PM1, and fog water and summarized them in Fig. 5. On average, Fresno PM1 exhibited a higher ratio compared to NYC (0.019 vs. 0.015), although both ratios were significantly lower than the ratio of fog organics (0.078). The mean NOON mass concentrations were estimated to be 70 ng m−3, 120 ng m−3, and 2.2 mg L−1 for the three sample sets, respectively. The ON levels observed in NYC and Fresno PM1 are relatively low compared to those observed in a forest site in southern China (0.2–1.1 µg m−3) (Yu et al., 2020) and in Hong Kong SAR (0.24 ± 0.09 µg m−3) (Li et al., 2022; Yu et al., 2021) but are similar to those observed in a recent study conducted in summertime in Nanjing, China (0.08–0.14 µg m−3) (Xian et al., 2023).
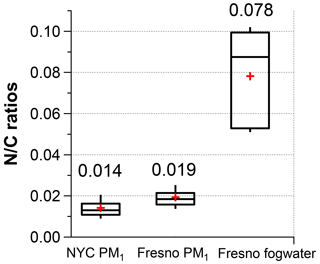
Figure 5 ratios of the organics in NYC PM1, Fresno PM1, and fog water (the whiskers above and below the boxes indicate the 90th and 10th percentiles, the upper and lower boundaries of the boxes indicate the 75th and 25th percentiles, the lines in the boxes indicate the median values, and the cross symbols indicate the mean values).
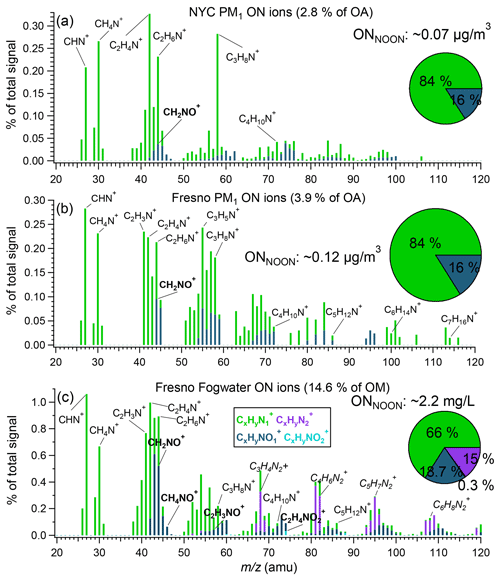
Figure 6Average mass spectra of ON ion categories (, , , and ) for NYC PM1 (a), Fresno PM1 (b), and Fresno fog water (c). The inset pie charts show the average ONNOON concentrations and mass contributions of the four ion categories (legends in c) to the total ONNOON, respectively.
The distribution of ON ions in NYC PM1, Fresno PM1, and fog water is further shown in Fig. 6. Across all spectra, ions dominated, accounting for 84 %, 84 %, and 66 % of the total NOON mass, respectively, indicating that amines are a major ON component in both cities. Notably, significant ions are observed, suggesting the presence of aliphatic amines, which aligns with previous findings (Zhang and Anastasio, 2003b, 2001). The presence of ions likely indicates the presence of amides.
Comparatively, the HR-AMS spectra of Fresno fog waters also exhibited prominent ions, likely originating from imidazoles formed through aqueous-phase reactions (De Haan et al., 2009a; De Haan et al., 2009b; Kim et al., 2019). In addition, appreciable signals of ( 74.0242), C2H3NO+ ( 57.0215), and CH4NO+ ( 46.0293), indicative of amino acids, are observed in fog water but are negligible in PM1, consistent with the findings reported in Zhang et al. (2002), where the quantification of amino compounds was achieved through derivatization high-performance liquid chromatography (HPLC) analysis. The enrichment of amino acids in fog water compared to PM1 can be attributed to a significant fraction of amino acids originating from proteinaceous matter, mainly associated with coarse-mode particles, such as soil dust and pollen. While these amino compounds may evade effective detection by the AMS in real time, they can be scavenged by the fog droplets.
In the preceding sections, we have explored the successful optimization of the HR-AMS methodology for the quantification and chemical characterization of NOON species in atmospheric aerosols and droplets. Nonetheless, it is important to acknowledge certain limitations associated with this method.
-
It is important to note that previous studies have shown bimodal size distributions of ON in marine aerosols (Violaki and Mihalopoulos, 2010; Cornell et al., 2001; Miyazaki et al., 2010), with a significant contribution from supermicron particles (PM>1). For example, the average concentrations of WSON were found to be 5.5 ± 3.9 and 11.6 ± 14.0 nmol m−3 for coarse (PM1.3–10) and fine (PM1.3) particles, respectively, at a remote marine location in the eastern Mediterranean (Violaki and Mihalopoulos, 2010). However, the HR-AMS has limitations in measuring ON in coarse particles in ambient air due to constraints in the transmission efficiency of the aerodynamic lens. As discussed earlier in Sect. 3.6, the difference between Fresno PM1 and co-collected fog water could, in part, be attributed to this limitation.
-
The AMS's thermal vaporization at ∼ 600 ∘C limits the detection of non-refractory species, potentially resulting in the underestimation of NOON levels if the amount of refractory N-containing species is significant. It is worth noting that the contribution of refractory ON to total ON is not yet well understood. Additionally, the precise quantification of NOON content faces challenges due to relatively significant uncertainties in the correction factor and RIE values. Furthermore, the 70 eV ionization process leads to extensive fragmentation, which adds complexity to the identification of individual NOON compounds.
-
The current method for analyzing liquid samples is mainly applicable to WSON. Future investigations may focus on using the HR-AMS to analyze the water-insoluble ON (WION), for example, by measuring samples extracted with organic solvents (Jiang et al., 2022; Chen et al., 2017).
-
The current method provides insights into ion fragments (fingerprint ions) associated with specific ON functional groups. However, the extensive fragmentation induced by the 70 eV electron ionization in the HR-AMS introduces inherent uncertainties in such identifications. For example, while a specific ion like CH4N+ likely originates from amines, we cannot rule out the possibility (albeit low) of its association with other ON types. In real atmospheric samples containing multiple ON varieties, the overlay and interference of specific ion fragments can be significant, increasing the level of uncertainty. To mitigate this challenge, the incorporation of data from soft ionization mass spectrometry techniques (Wang et al., 2019; Song et al., 2022; Mao et al., 2022) alongside HR-AMS data can significantly enhance the analyses of NOON species, thereby reducing uncertainties and advancing our understanding of their composition.
This study focuses on the development of methodologies using the HR-AMS for quantification and characterization of NOON species in ambient aerosols and aqueous droplets. Through extensive analysis of HR-AMS spectra from 75 NOON standards, we reach several important conclusions. Firstly, we show that NOON compounds can generate significant and ion fragments, which are commonly assigned to inorganic nitrogen species in ambient AMS analysis. Additionally, although the presence of CH2N+ ( = 28.0187) is common in the AMS spectra of NOON compounds, it is often overlooked due to interference from air-related in ambient datasets. Secondly, the average RIE for NOON was determined to be 1.52 (± 0.58), which aligns well with the default RIE value of 1.4 for total OA quantification. By considering these factors, we recommend a new calibration factor of 0.79 for NOON quantification.
Furthermore, we examined the HRMS of various NOON compounds to identify chemical fingerprint ions that can aid in the NOON speciation in ambient HR-AMS datasets. Our findings reveal distinct ion patterns associated with different NOON types. Specifically, ions serve as tracer ions for amines (particularly CH4N+, C2H6N+, and C3H8N+ are markers of primary, secondary, and tertiary amines, respectively). The presence of CnH2nNO+ ions (especially for n = 1–4) is a strong signal for the presence of amides. Tracer ions for amino acids include , C2H3NO+, and CH4NO+. The ion series are characteristic of 2N-heterocyclic compounds. It is worth noting that ion can be produced from amines and amino acids but not from inorganic nitrogen species. Additionally, we compared the HR-AMS and NIST database spectra for the NOON species. The level of similarity varied significantly among different types. Pearson's correlation coefficients (r2) for amines, amides, N heterocycles, nitro compounds, and amino acids were found to be 0.70, 0.63, 0.57, 0.50, and 0.23, respectively.
Lastly, we applied the methodologies described above to analyze three HR-AMS datasets, which included online measurements of PM1 and offline analysis of fog water. The fog water in Fresno exhibited significantly higher ratios (average = 0.078) compared to the PM1 samples (0.019 in Fresno and 0.015 in NYC). Regarding NOON constitution, both Fresno and NYC PM1 contained significant amounts of amino compounds, whereas Fresno fog water exhibited a broader range of NOON species, including N-containing aromatic heterocycles (e.g., imidazoles) and amides.
Moving forward, our aim is to extend this approach to multiple HR-AMS datasets collected from diverse locations worldwide. By doing so, we anticipate gaining a more comprehensive understanding of the chemical characteristics, sources, and processes of atmospheric ON. This broader application will contribute to advancing our knowledge regarding airborne organic nitrogen compounds and their role in atmospheric chemistry on a global scale.
All datasets, including HR-AMS mass spectra, are available upon request from Qi Zhang (dkwzhang@ucdavis.edu.cn) and Xinlei Ge (caxinra@163.com).
The supplement related to this article is available online at: https://doi.org/10.5194/amt-17-423-2024-supplement.
QZ conceived and designed the experiments, while XG and YS conducted the experiments. XG, YS, JT, and QZ analyzed the HR-AMS spectra of the standards and the ambient data. XG and QZ wrote the paper with input from all authors.
The contact author has declared that none of the authors has any competing interests.
Publisher's note: Copernicus Publications remains neutral with regard to jurisdictional claims made in the text, published maps, institutional affiliations, or any other geographical representation in this paper. While Copernicus Publications makes every effort to include appropriate place names, the final responsibility lies with the authors.
The authors acknowledge the financial support of the U.S. Department of Energy Office of Science Atmospheric System Research Program, the U.S. National Institute of Environmental Health Sciences Core Center, the Natural Science Foundation of China, and the Jiangsu Natural Science Foundation.
This research has been supported by the U.S. Department of Energy (grant no. DE-SC0022140), the National Institute of Environmental Health Sciences (grant no. P30 ES023513), the National Natural Science Foundation of China (grant nos. 42021004 and 21976093), and the Natural Science Foundation of Jiangsu Province (grant no. BK20150042).
This paper was edited by Meng Gao and reviewed by four anonymous referees.
Aiken, A. C., DeCarlo, P. F., and Jimenez, J. L.: Elemental analysis of organic species with electron ionization high-resolution mass spectrometry, Anal. Chem., 79, 8350–8358, https://doi.org/10.1021/ac071150w, 2007.
Aiken, A. C., Decarlo, P. F., Kroll, J. H., Worsnop, D. R., Huffman, J. A., Docherty, K. S., Ulbrich, I. M., Mohr, C., Kimmel, J. R., Sueper, D., Sun, Y., Zhang, Q., Trimborn, A., Northway, M., Ziemann, P. J., Canagaratna, M. R., Onasch, T. B., Alfarra, M. R., Prevot, A. S. H., Dommen, J., Duplissy, J., Metzger, A., Baltensperger, U., and Jimenez, J. L.: and ratios of primary, secondary, and ambient organic aerosols with high-resolution time-of-flight aerosol mass spectrometry, Environ. Sci. Technol., 42, 4478–4485, https://doi.org/10.1021/es703009q, 2008.
Akyüz, M.: Simultaneous determination of aliphatic and aromatic amines in indoor and outdoor air samples by gas chromatography–mass spectrometry, Talanta, 71, 486–492, https://doi.org/10.1016/j.talanta.2006.10.028, 2007.
Almeida, J., Schobesberger, S., Kurten, A., Ortega, I. K., Kupiainen-Maatta, O., Praplan, A. P., Adamov, A., Amorim, A., Bianchi, F., Breitenlechner, M., David, A., Dommen, J., Donahue, N. M., Downard, A., Dunne, E., Duplissy, J., Ehrhart, S., Flagan, R. C., Franchin, A., Guida, R., Hakala, J., Hansel, A., Heinritzi, M., Henschel, H., Jokinen, T., Junninen, H., Kajos, M., Kangasluoma, J., Keskinen, H., Kupc, A., Kurten, T., Kvashin, A. N., Laaksonen, A., Lehtipalo, K., Leiminger, M., Leppa, J., Loukonen, V., Makhmutov, V., Mathot, S., McGrath, M. J., Nieminen, T., Olenius, T., Onnela, A., Petaja, T., Riccobono, F., Riipinen, I., Rissanen, M., Rondo, L., Ruuskanen, T., Santos, F. D., Sarnela, N., Schallhart, S., Schnitzhofer, R., Seinfeld, J. H., Simon, M., Sipila, M., Stozhkov, Y., Stratmann, F., Tome, A., Trostl, J., Tsagkogeorgas, G., Vaattovaara, P., Viisanen, Y., Virtanen, A., Vrtala, A., Wagner, P. E., Weingartner, E., Wex, H., Williamson, C., Wimmer, D., Ye, P., Yli-Juuti, T., Carslaw, K. S., Kulmala, M., Curtius, J., Baltensperger, U., Worsnop, D. R., Vehkamaki, H., and Kirkby, J.: Molecular understanding of sulphuric acid-amine particle nucleation in the atmosphere, Nature, 502, 359–363, https://doi.org/10.1038/nature12663, 2013.
Altieri, K. E., Hastings, M. G., Peters, A. J., and Sigman, D. M.: Molecular characterization of water soluble organic nitrogen in marine rainwater by ultra-high resolution electrospray ionization mass spectrometry, Atmos. Chem. Phys., 12, 3557–3571, https://doi.org/10.5194/acp-12-3557-2012, 2012.
Bruns, E. A., Perraud, V., Zelenyuk, A., Ezell, M. J., Johnson, S. N., Yu, Y., Imre, D., Finlayson-Pitts, B. J., and Alexander, M. L.: Comparison of FTIR and Particle Mass Spectrometry for the Measurement of Particulate Organic Nitrates, Environ. Sci. Technol., 44, 1056–1061, https://doi.org/10.1021/es9029864, 2010.
Canagaratna, M. R., Jayne, J. T., Jimenez, J. L., Allan, J. D., Alfarra, M. R., Zhang, Q., Onasch, T. B., Drewnick, F., Coe, H., Middlebrook, A., Delia, A., Williams, L. R., Trimborn, A. M., Northway, M. J., DeCarlo, P. F., Kolb, C. E., Davidovits, P., and Worsnop, D. R.: Chemical and microphysical characterization of ambient aerosols with the aerodyne aerosol mass spectrometer, Mass Spectrom. Rev., 26, 185–222, https://doi.org/10.1002/Mas.20115, 2007.
Canagaratna, M. R., Jimenez, J. L., Kroll, J. H., Chen, Q., Kessler, S. H., Massoli, P., Hildebrandt Ruiz, L., Fortner, E., Williams, L. R., Wilson, K. R., Surratt, J. D., Donahue, N. M., Jayne, J. T., and Worsnop, D. R.: Elemental ratio measurements of organic compounds using aerosol mass spectrometry: characterization, improved calibration, and implications, Atmos. Chem. Phys., 15, 253–272, https://doi.org/10.5194/acp-15-253-2015, 2015.
Cape, J. N., Cornell, S. E., Jickells, T. D., and Nemitz, E.: Organic nitrogen in the atmosphere — Where does it come from? A review of sources and methods, Atmos. Res., 102, 30–48, https://doi.org/10.1016/j.atmosres.2011.07.009, 2011.
Chen, Q., Ikemori, F., Nakamura, Y., Vodicka, P., Kawamura, K., and Mochida, M.: Structural and light-absorption characteristics of complex water-insoluble organic mixtures in urban submicrometer aerosols, Environ. Sci. Technol., 51, 8293–8303, https://doi.org/10.1021/acs.est.7b01630, 2017.
Cornell, S., Randell, A., and Jickells, T.: Atmospheric inputs of dissolved organic nitrogen to the oceans, Nature, 376, 243–246, https://doi.org/10.1038/376243a0, 1995.
Cornell, S., Mace, K., Coeppicus, S., Duce, R., Huebert, B., Jickells, T., and Zhuang, L. Z.: Organic nitrogen in Hawaiian rain and aerosol, J. Geophys. Res.-Atmos., 106, 7973–7983, https://doi.org/10.1029/2000jd900655, 2001.
Cornell, S., Jickells, T. D., Cape, J. N., Rowland, A. P., and Duce, R. A.: Organic nitrogen deposition on land and coastal environments: a review of methods and data, Atmos. Environ., 37, 2173–2191, https://doi.org/10.1016/S1352-2310(03)00133-X, 2003.
Day, D. A., Campuzano-Jost, P., Nault, B. A., Palm, B. B., Hu, W., Guo, H., Wooldridge, P. J., Cohen, R. C., Docherty, K. S., Huffman, J. A., de Sá, S. S., Martin, S. T., and Jimenez, J. L.: A systematic re-evaluation of methods for quantification of bulk particle-phase organic nitrates using real-time aerosol mass spectrometry, Atmos. Meas. Tech., 15, 459–483, https://doi.org/10.5194/amt-15-459-2022, 2022.
De Haan, D. O., Tolbert, M. A., and Jimenez, J. L.: Atmospheric condensed-phase reactions of glyoxal with methylamine, Geophys. Res. Lett., 36, L11819, https://doi.org/10.1029/2009gl037441, 2009a.
De Haan, D. O., Corrigan, A. L., Smith, K. W., Stroik, D. R., Turley, J. J., Lee, F. E., Tolbert, M. A., Jimenez, J. L., Cordova, K. E., and Ferrell, G. R.: Secondary organic aerosol-forming reactions of glyoxal with amino acids, Environ. Sci. Technol., 43, 2818–2824, https://doi.org/10.1021/es803534f, 2009b.
DeCarlo, P. F., Kimmel, J. R., Trimborn, A., Northway, M. J., Jayne, J. T., Aiken, A. C., Gonin, M., Fuhrer, K., Horvath, T., Docherty, K. S., Worsnop, D. R., and Jimenez, J. L.: Field-deployable, high-resolution, time-of-flight aerosol mass spectrometer, Anal. Chem., 78, 8281–8289, https://doi.org/10.1021/ac061249n, 2006.
Docherty, K. S., Lewandowski, M., and Jimenez, J. L.: Effect of vaporizer temperature on ambient non-refractory submicron aerosol composition and mass spectra measured by the aerosol mass spectrometer, Aerosol Sci. Technol., 49, 485–494, https://doi.org/10.1080/02786826.2015.1042100, 2015.
Drewnick, F.: Speciation analysis in on-line aerosol mass spectrometry, Anal. Bioanal. Chem., 404, 2127–2131, https://doi.org/10.1007/s00216-012-6295-x, 2012.
Farmer, D. K., Matsunaga, A., Docherty, K. S., Surratt, J. D., Seinfeld, J. H., Ziemann, P. J., and Jimenez, J. L.: Response of an aerosol mass spectrometer to organonitrates and organosulfates and implications for atmospheric chemistry, P. Natl. Acad. Sci. USA, 107, 6670–6675, https://doi.org/10.1073/pnas.0912340107, 2010.
Fry, J. L., Kiendler-Scharr, A., Rollins, A. W., Wooldridge, P. J., Brown, S. S., Fuchs, H., Dubé, W., Mensah, A., dal Maso, M., Tillmann, R., Dorn, H.-P., Brauers, T., and Cohen, R. C.: Organic nitrate and secondary organic aerosol yield from NO3 oxidation of β-pinene evaluated using a gas-phase kinetics/aerosol partitioning model, Atmos. Chem. Phys., 9, 1431–1449, https://doi.org/10.5194/acp-9-1431-2009, 2009.
Ge, X., Wexler, A. S., and Clegg, S. L.: Atmospheric amines – Part I. A review, Atmos. Environ., 45, 524–546, https://doi.org/10.1016/j.atmosenv.2010.10.012, 2011a.
Ge, X., Wexler, A. S., and Clegg, S. L.: Atmospheric amines - Part II. Thermodynamic properties and gas/particle partitioning, Atmos. Environ., 45, 561–577, https://doi.org/10.1016/j.atmosenv.2010.10.013, 2011b.
Ge, X., Setyan, A., Sun, Y., and Zhang, Q.: Primary and secondary organic aerosols in Fresno, California during wintertime: Results from high resolution aerosol mass spectrometry, J. Geophys. Res.-Atmos., 117, D19301, https://doi.org/10.1029/2012jd018026, 2012a.
Ge, X., Zhang, Q., Sun, Y., Ruehl, C. R., and Setyan, A.: Effect of aqueous-phase processing on aerosol chemistry and size distributions in Fresno, California, during wintertime, Environ. Chem., 9, 221–235, https://doi.org/10.1071/EN11168, 2012b.
Ge, X., Shaw, S. L., and Zhang, Q.: Toward understanding amines and their degradation products from postcombustion CO2 capture processes with aerosol mass spectrometry, Environ. Sci. Technol., 48, 5066–5075, https://doi.org/10.1021/es4056966, 2014.
Ge, X., Li, L., Chen, Y., Chen, H., Wu, D., Wang, J., Xie, X., Ge, S., Ye, Z., Xu, J., and Chen, M.: Aerosol characteristics and sources in Yangzhou, China resolved by offline aerosol mass spectrometry and other techniques, Environ. Pollut., 225, 74–85, https://doi.org/10.1016/j.envpol.2017.03.044, 2017.
Hawkins, L. N., Welsh, H. G., and Alexander, M. V.: Evidence for pyrazine-based chromophores in cloud water mimics containing methylglyoxal and ammonium sulfate, Atmos. Chem. Phys., 18, 12413–12431, https://doi.org/10.5194/acp-18-12413-2018, 2018.
Herckes, P., Leenheer, J. A., and Jeffrey L. Collett, J.: Comprehensive characterization of atmospheric organic matter in Fresno, California fog water, Environ. Sci. Technol., 41, 393–399, https://doi.org/10.1021/es0607988, 2007.
Huang, W., Yang, Y., Wang, Y., Gao, W., Li, H., Zhang, Y., Li, J., Zhao, S., Yan, Y., Ji, D., Tang, G., Liu, Z., Wang, L., Zhang, R., and Wang, Y.: Exploring the inorganic and organic nitrate aerosol formation regimes at a suburban site on the North China Plain, Sci. Total Environ., 768, 144538, https://doi.org/10.1016/j.scitotenv.2020.144538, 2021.
Huang, Y., Chen, H., Wang, L., Yang, X., and Chen, J. M.: Single particle analysis of amines in ambient aerosol in Shanghai, Environ. Chem., 9, 202–210, https://doi.org/10.1071/en11145, 2012.
Jiang, X., Liu, D., Li, Q., Tian, P., Wu, Y., Li, S., Hu, K., Ding, S., Bi, K., Li, R., Huang, M., Ding, D., Chen, Q., Kong, S., Li, W., Pang, Y., and He, D.: Connecting the light absorption of atmospheric organic aerosols with oxidation state and polarity, Environ. Sci. Technol., 56, 12873–12885, https://doi.org/10.1021/acs.est.2c02202, 2022.
Jickells, T., Baker, A. R., Cape, J. N., Cornell, S. E., and Nemitz, E.: The cycling of organic nitrogen through the atmosphere, Philos. T. R. Soc. B., 368, https://doi.org/10.1098/rstb.2013.0115, 2013.
Junninen, H., Ehn, M., Petäjä, T., Luosujärvi, L., Kotiaho, T., Kostiainen, R., Rohner, U., Gonin, M., Fuhrer, K., Kulmala, M., and Worsnop, D. R.: A high-resolution mass spectrometer to measure atmospheric ion composition, Atmos. Meas. Tech., 3, 1039–1053, https://doi.org/10.5194/amt-3-1039-2010, 2010.
Kanakidou, M., Myriokefalitakis, S., Daskalakis, N., Fanourgakis, G., Nenes, A., Baker, A. R., Tsigaridis, K., and Mihalopoulos, N.: Past, present, and future atmospheric nitrogen deposition, J. Atmos. Sci., 73, 2039–2047, https://doi.org/10.1175/JAS-D-15-0278.1, 2016.
Kim, H., Collier, S., Ge, X., Xu, J., Sun, Y., Jiang, W., Wang, Y., Herckes, P., and Zhang, Q.: Chemical processing of water-soluble species and formation of secondary organic aerosol in fogs, Atmos. Environ., 200, 158–166, https://doi.org/10.1016/j.atmosenv.2018.11.062, 2019.
Laskin, A., Smith, J. S., and Laskin, J.: Molecular characterization of nitrogen-containing organic compounds in biomass burning aerosols using high-resolution mass spectrometry, Environ. Sci. Technol., 43, 3764–3771, https://doi.org/10.1021/es803456n, 2009.
Lee, A. K. Y., Zhao, R., Li, R., Liggio, J., Li, S.-M., and Abbatt, J. P. D.: Formation of light absorbing organo-nitrogen species from evaporation of droplets containing glyoxal and ammonium sulfate, Environ. Sci. Technol., 47, 12819–12826, https://doi.org/10.1021/es402687w, 2013.
Li, J., Yu, X., Li, Q., Wang, S., Cheng, Y. Y., and Yu, J. Z.: Online measurement of aerosol inorganic and organic nitrogen based on thermal evolution and chemiluminescent detection, Atmos. Environ., 271, 118905, https://doi.org/10.1016/j.atmosenv.2021.118905, 2022.
Li, K., Sinha, B., and Hoppe, P.: Speciation of nitrogen-bearing species using negative and positive secondary ion spectra with nano secondary ion mass spectrometry, Anal. Chem., 88, 3281–3288, https://doi.org/10.1021/acs.analchem.5b04740, 2016.
Lin, C., Huang, R.-J., Duan, J., Zhong, H., and Xu, W.: Primary and secondary organic nitrate in northwest China: A case study, Environ. Sci. Tech. Let., 8, 947–953, https://doi.org/10.1021/acs.estlett.1c00692, 2021.
Liu, F., Bi, X., Zhang, G., Peng, L., Lian, X., Lu, H., Fu, Y., Wang, X., Peng, P. a., and Sheng, G.: Concentration, size distribution and dry deposition of amines in atmospheric particles of urban Guangzhou, China, Atmos. Environ., 171, 279–288, https://doi.org/10.1016/j.atmosenv.2017.10.016, 2017.
Liu, Y., Li, H., Cui, S., Nie, D., Chen, Y., and Ge, X.: Chemical characteristics and sources of water-soluble organic nitrogen species in PM2.5 in Nanjing, China, Atmosphere, 12, 574, https://doi.org/10.3390/atmos12050574, 2021.
Ma, M., Rivellini, L.-H., Cui, Y., Willis, M. D., Wilkie, R., Abbatt, J. P. D., Canagaratna, M. R., Wang, J., Ge, X., and Lee, A. K. Y.: Elemental analysis of oxygenated organic coating on black carbon particles using a soot-particle aerosol mass spectrometer, Atmos. Meas. Tech., 14, 2799–2812, https://doi.org/10.5194/amt-14-2799-2021, 2021.
Mao, J., Cheng, Y., Bai, Z., Zhang, W., Zhang, L., Chen, H., Wang, L., Li, L., and Chen, J.: Molecular characterization of nitrogen-containing organic compounds in the winter North China Plain, Sci. Total Environ., 838, 156189, https://doi.org/10.1016/j.scitotenv.2022.156189, 2022.
McLafferty, F. W. and Turecek, F.: Interpretation of Mass Spectra, University Science Books, Mill Valley, California, ISBN-10 978-0-935702-25-5, 1993.
Miyazaki, Y., Kawamura, K., and Sawano, M.: Size distributions of organic nitrogen and carbon in remote marine aerosols: Evidence of marine biological origin based on their isotopic ratios, Geophys. Res. Lett., 37, L06803, https://doi.org/10.1029/2010GL042483, 2010.
Miyazaki, Y., Kawamura, K., Jung, J., Furutani, H., and Uematsu, M.: Latitudinal distributions of organic nitrogen and organic carbon in marine aerosols over the western North Pacific, Atmos. Chem. Phys., 11, 3037–3049, https://doi.org/10.5194/acp-11-3037-2011, 2011.
Murphy, S. M., Sorooshian, A., Kroll, J. H., Ng, N. L., Chhabra, P., Tong, C., Surratt, J. D., Knipping, E., Flagan, R. C., and Seinfeld, J. H.: Secondary aerosol formation from atmospheric reactions of aliphatic amines, Atmos. Chem. Phys., 7, 2313–2337, https://doi.org/10.5194/acp-7-2313-2007, 2007.
Niedek, C. R., Mei, F., Zawadowicz, M. A., Zhu, Z., Schmid, B., and Zhang, Q.: Quantitative chemical assay of nanogram-level particulate matter using aerosol mass spectrometry: characterization of particles collected from uncrewed atmospheric measurement platforms, Atmos. Meas. Tech., 16, 955–968, https://doi.org/10.5194/amt-16-955-2023, 2023.
O'Brien, R. E., Ridley, K. J., Canagaratna, M. R., Jayne, J. T., Croteau, P. L., Worsnop, D. R., Budisulistiorini, S. H., Surratt, J. D., Follett, C. L., Repeta, D. J., and Kroll, J. H.: Ultrasonic nebulization for the elemental analysis of microgram-level samples with offline aerosol mass spectrometry, Atmos. Meas. Tech., 12, 1659–1671, https://doi.org/10.5194/amt-12-1659-2019, 2019.
Özel, M. Z., Ward, M. W., Hamilton, J. F., Lewis, A. C., Raventós-Duran, T., and Harrison, R. M.: Analysis of organic nitrogen compounds in urban aerosol samples using GCxGC-TOF/MS, Aerosol Sci. Technol., 44, 109–116, https://doi.org/10.1080/02786820903410105, 2009.
Özel, M. Z., Hamilton, J. F., and Lewis, A. C.: New sensitive and quantitative analysis method for organic nitrogen compounds in urban aerosol samples, Environ. Sci. Technol., 45, 1497–1505, https://doi.org/10.1021/es102528g, 2011.
Parworth, C. L., Young, D. E., Kim, H., Zhang, X., Cappa, C. D., Collier, S., and Zhang, Q.: Wintertime water-soluble aerosol composition and particle water content in Fresno, California, J. Geophys. Res.-Atmos., 122, 3155–3170, https://doi.org/10.1002/2016JD026173, 2017.
Place, B. K., Quilty, A. T., Di Lorenzo, R. A., Ziegler, S. E., and VandenBoer, T. C.: Quantitation of 11 alkylamines in atmospheric samples: separating structural isomers by ion chromatography, Atmos. Meas. Tech., 10, 1061–1078, https://doi.org/10.5194/amt-10-1061-2017, 2017.
Powelson, M. H., Espelien, B. M., Hawkins, L. N., Galloway, M. M., and De Haan, D. O.: Brown carbon formation by aqueous-phase carbonyl compound reactions with amines and ammonium sulfate, Environ. Sci. Technol., 48, 985–993, https://doi.org/10.1021/es4038325, 2014.
Price, D. J., Piasecki, A. M., Shah, R. U., Hayden, K. L., Burkholder, J. B., Roberts, J. M., and Middlebrook, A. M.: Reactive nitrogen and total organic carbon calibration techniques for the Aerodyne aerosol mass spectrometer, Aerosol Sci. Technol., 57, 727–741, https://doi.org/10.1080/02786826.2023.2218462, 2023.
Qi, L., Bozzetti, C., Corbin, J. C., Daellenbach, K. R., El Haddad, I., Zhang, Q., Wang, J., Baltensperger, U., Prévôt, A. S. H., Chen, M., Ge, X., and Slowik, J. G.: Source identification and characterization of organic nitrogen in atmospheric aerosols at a suburban site in China, Sci. Total Environ., 818, 151800, https://doi.org/10.1016/j.scitotenv.2021.151800, 2022.
Rincon, A. G., Calvo, A. I., Dietzel, M., and Kalberer, M.: Seasonal differences of urban organic aerosol composition – an ultra-high resolution mass spectrometry study, Environ. Chem., 9, 298–319, https://doi.org/10.1071/EN12016, 2012.
Rovelli, G., Miles, R. E. H., Reid, J. P., and Clegg, S. L.: Hygroscopic properties of aminium sulfate aerosols, Atmos. Chem. Phys., 17, 4369–4385, https://doi.org/10.5194/acp-17-4369-2017, 2017.
Ruiz-Jimenez, J., Hautala, S., Parshintsev, J., Laitinen, T., Hartonen, K., Petaja, T., Kulmala, M., and Riekkola, M. L.: Aliphatic and aromatic amines in atmospheric aerosol particles: Comparison of three ionization techniques in liquid chromatography-mass spectrometry and method development, Talanta, 97, 55–62, https://doi.org/10.1016/j.talanta.2012.03.062, 2012.
Russell, K. M., Keene, W. C., Maben, J. R., Galloway, J. N., and Moody, J. L.: Phase partitioning and dry deposition of atmospheric nitrogen at the mid-Atlantic U. S. coast, J. Geophys. Res.-Atmos., 108, 4656, https://doi.org/10.1029/2003JD003736, 2003.
Samy, S. and Hays, M. D.: Quantitative LC–MS for water-soluble heterocyclic amines in fine aerosols (PM2.5) at Duke Forest, USA, Atmos. Environ., 72, 77–80, https://doi.org/10.1016/j.atmosenv.2013.02.032, 2013.
Samy, S., Robinson, J., and Hays, M. D.: An advanced LC-MS (Q-TOF) technique for the detection of amino acids in atmospheric aerosols, Anal. Bioanal. Chem., 401, 3103–3113, https://doi.org/10.1007/s00216-011-5238-2, 2011.
Setyan, A., Song, C., Merkel, M., Knighton, W. B., Onasch, T. B., Canagaratna, M. R., Worsnop, D. R., Wiedensohler, A., Shilling, J. E., and Zhang, Q.: Chemistry of new particle growth in mixed urban and biogenic emissions – insights from CARES, Atmos. Chem. Phys., 14, 6477–6494, https://doi.org/10.5194/acp-14-6477-2014, 2014.
Shi, X., Qiu, X., Cheng, Z., Chen, Q., Rudich, Y., and Zhu, T.: Isomeric identification of particle-phase organic nitrates through gas chromatography and time-of-flight mass spectrometry coupled with an electron capture negative ionization source, Environ. Sci. Technol., 54, 707–713, https://doi.org/10.1021/acs.est.9b05818, 2020.
Smith, J. N., Barsanti, K. C., Friedli, H. R., Ehn, M., Kulmala, M., Collins, D. R., Scheckman, J. H., Williams, B. J., and McMurry, P. H.: Observations of aminium salts in atmospheric nanoparticles and possible climatic implications, P. Natl. Acad. Sci. USA, 107, 6634–6639, https://doi.org/10.1073/pnas.0912127107, 2010.
Song, J., Li, M., Zou, C., Cao, T., Fan, X., Jiang, B., Yu, Z., Jia, W., and Peng, P. a.: Molecular characterization of nitrogen-containing compounds in humic-like substances emitted from biomass burning and coal combustion, Environ. Sci. Technol., 56, 119–130, https://doi.org/10.1021/acs.est.1c04451, 2022.
Song, T., Wang, S., Zhang, Y., Song, J., Liu, F., Fu, P., Shiraiwa, M., Xie, Z., Yue, D., Zhong, L., Zheng, J., and Lai, S.: Proteins and amino acids in fine particulate matter in rural Guangzhou, southern China: Seasonal cycles, sources, and atmospheric processes, Environ. Sci. Technol., 51, 6773–6781, https://doi.org/10.1021/acs.est.7b00987, 2017.
Sun, Y. L., Zhang, Q., Anastasio, C., and Sun, J.: Insights into secondary organic aerosol formed via aqueous-phase reactions of phenolic compounds based on high resolution mass spectrometry, Atmos. Chem. Phys., 10, 4809–4822, https://doi.org/10.5194/acp-10-4809-2010, 2010.
Sun, Y.-L., Zhang, Q., Schwab, J. J., Demerjian, K. L., Chen, W.-N., Bae, M.-S., Hung, H.-M., Hogrefe, O., Frank, B., Rattigan, O. V., and Lin, Y.-C.: Characterization of the sources and processes of organic and inorganic aerosols in New York city with a high-resolution time-of-flight aerosol mass apectrometer, Atmos. Chem. Phys., 11, 1581–1602, https://doi.org/10.5194/acp-11-1581-2011, 2011.
VandenBoer, T. C., Petroff, A., Markovic, M. Z., and Murphy, J. G.: Size distribution of alkyl amines in continental particulate matter and their online detection in the gas and particle phase, Atmos. Chem. Phys., 11, 4319–4332, https://doi.org/10.5194/acp-11-4319-2011, 2011.
Verriele, M., Plaisance, H., Depelchin, L., Benchabane, S., Locoge, N., and Meunier, G.: Determination of 14 amines in air samples using midget impingers sampling followed by analysis with ion chromatography in tandem with mass spectrometry, J. Environ. Monitor., 14, 402–408, https://doi.org/10.1039/C2EM10636A, 2012.
Violaki, K. and Mihalopoulos, N.: Water-soluble organic nitrogen (WSON) in size-segregated atmospheric particles over the Eastern Mediterranean, Atmos. Environ., 44, 4339–4345 https://doi.org/10.1016/j.atmosenv.2010.07.056, 2010.
Wang, Y., Hu, M., Lin, P., Tan, T., Li, M., Xu, N., Zheng, J., Du, Z., Qin, Y., Wu, Y., Lu, S., Song, Y., Wu, Z., Guo, S., Zeng, L., Huang, X., and He, L.: Enhancement in particulate organic nitrogen and light absorption of humic-like substances over Tibetan plateau due to long-range transported biomass burning emissions, Environ. Sci. Technol., 53, 14222–14232, https://doi.org/10.1021/acs.est.9b06152, 2019.
Xian, J., Cui, S., Chen, X., Wang, J., Xiong, Y., Gu, C., Wang, Y., Zhang, Y., Li, H., Wang, J., and Ge, X.: Online chemical characterization of atmospheric fine secondary aerosols and organic nitrates in summer Nanjing, China, Atmos. Res., 290, 106783, https://doi.org/10.1016/j.atmosres.2023.106783, 2023.
Xu, W., Sun, Y., Wang, Q., Du, W., Zhao, J., Ge, X., Han, T., Zhang, Y., Zhou, W., Li, J., Fu, P., Wang, Z., and Worsnop, D. R.: Seasonal characterization of organic nitrogen in atmospheric aerosols using high resolution aerosol mass spectrometry in Beijing, China, ACS Earth Space Chem., 1, 673–682, https://doi.org/10.1021/acsearthspacechem.7b00106, 2017.
Yao, L., Wang, M.-Y., Wang, X.-K., Liu, Y.-J., Chen, H.-F., Zheng, J., Nie, W., Ding, A.-J., Geng, F.-H., Wang, D.-F., Chen, J.-M., Worsnop, D. R., and Wang, L.: Detection of atmospheric gaseous amines and amides by a high-resolution time-of-flight chemical ionization mass spectrometer with protonated ethanol reagent ions, Atmos. Chem. Phys., 16, 14527–14543, https://doi.org/10.5194/acp-16-14527-2016, 2016.
Yao, L., Garmash, O., Bianchi, F., Zheng, J., Yan, C., Kontkanen, J., Junninen, H., Mazon, S. B., Ehn, M., Paasonen, P., Sipilä, M., Wang, M., Wang, X., Xiao, S., Chen, H., Lu, Y., Zhang, B., Wang, D., Fu, Q., Geng, F., Li, L., Wang, H., Qiao, L., Yang, X., Chen, J., Kerminen, V.-M., Petäjä, T., Worsnop, D. R., Kulmala, M., and Wang, L.: Atmospheric new particle formation from sulfuric acid and amines in a Chinese megacity, Science, 361, 278–281, https://doi.org/10.1126/science.aao4839, 2018.
Ye, C., Zhang, N., Gao, H., and Zhou, X.: Photolysis of particulate nitrate as a source of HONO and NOx, Environ. Sci. Technol., 51, 6849–6856, https://doi.org/10.1021/acs.est.7b00387, 2017.
Yu, H. and Lee, S.-H.: Chemical ionisation mass spectrometry for the measurement of atmospheric amines, Environ. Chem., 9, 190–201, https://doi.org/10.1071/EN12020, 2012.
Yu, K., Zhu, Q., Du, K., and Huang, X.-F.: Characterization of nighttime formation of particulate organic nitrates based on high-resolution aerosol mass spectrometry in an urban atmosphere in China, Atmos. Chem. Phys., 19, 5235–5249, https://doi.org/10.5194/acp-19-5235-2019, 2019.
Yu, X., Yu, Q., Zhu, M., Tang, M., Li, S., Yang, W., Zhang, Y., Deng, W., Li, G., Yu, Y., Huang, Z., Song, W., Ding, X., Hu, Q., Li, J., Bi, X., and Wang, X.: Water soluble organic nitrogen (WSON) in ambient fine particles over a megacity in south China: Spatiotemporal variations and source apportionment, J. Geophys. Res.-Atmos., 122, 13045–13060, https://doi.org/10.1002/2017JD027327, 2017.
Yu, X., Li, D., Li, D., Zhang, G., Zhou, H., Li, S., Song, W., Zhang, Y., Bi, X., Yu, J., and Wang, X.: Enhanced wet deposition of water-soluble organic nitrogen during the harvest season: Influence of biomass burning and in-cloud scavenging, J. Geophys. Res.-Atmos., 125, e2020JD032699, https://doi.org/10.1029/2020JD032699, 2020.
Yu, X., Li, Q., Ge, Y., Li, Y., Liao, K., Huang, X. H., Li, J., and Yu, J. Z.: Simultaneous determination of aerosol inorganic and organic nitrogen by thermal evolution and chemiluminescence detection, Environ. Sci. Technol., 55, 11579–11589, https://doi.org/10.1021/acs.est.1c04876, 2021.
Zhang, Q. and Anastasio, C.: Chemistry of fog waters in California's Central Valley – Part 3: concentrations and speciation of organic and inorganic nitrogen, Atmos. Environ., 35, 5629–5643, https://doi.org/10.1016/S1352-2310(01)00337-5, 2001.
Zhang, Q. and Anastasio, C.: Conversion of fogwater and aerosol organic nitrogen to ammonium, nitrate, and NOx during exposure to simulated sunlight and ozone, Environ. Sci. Technol., 37, 3522–3530, https://doi.org/10.1021/es034114x, 2003a.
Zhang, Q. and Anastasio, C.: Free and combined amino compounds in atmospheric fine particles (PM2.5) and fog waters from Northern California, Atmos. Environ., 37, 2247–2258, https://doi.org/10.1016/s1352-2310(03)00127-4, 2003b.
Zhang, Q., Anastasio, C., and Jimemez-Cruz, M.: Water-soluble organic nitrogen in atmospheric fine particles (PM2.5) from northern California, J. Geophys. Res.-Atmos., 107, AAC 3-1–AAC 3-9, https://doi.org/10.1029/2001jd000870, 2002.
Zhang, Y., Wang, J., Cui, S., Huang, D. D., and Ge, X.: Aerosol measurements by soot particle aerosol mass spectrometer: A review, Curr. Pollut. Rep., 6, 440–451, https://doi.org/10.1007/s40726-020-00162-4, 2020.
Zheng, J., Ma, Y., Chen, M., Zhang, Q., Wang, L., Khalizov, A. F., Yao, L., Wang, Z., Wang, X., and Chen, L.: Measurement of atmospheric amines and ammonia using the high resolution time-of-flight chemical ionization mass spectrometry, Atmos. Environ., 102, 249–259, https://doi.org/10.1016/j.atmosenv.2014.12.002, 2015.
Zhou, S., Collier, S., Xu, J., Mei, F., Wang, J., Lee, Y.-N., Sedlacek, A. J., Springston, S. R., Sun, Y., and Zhang, Q.: Influences of upwind emission sources and atmospheric processing on aerosol chemistry and properties at a rural location in the Northeastern U.S, J. Geophys. Res.-Atmos., 121, 2015JD024568, https://doi.org/10.1002/2015JD024568, 2016.
Zhu, Q., He, L.-Y., Huang, X.-F., Cao, L.-M., Gong, Z.-H., Wang, C., Zhuang, X., and Hu, M.: Atmospheric aerosol compositions and sources at two national background sites in northern and southern China, Atmos. Chem. Phys., 16, 10283–10297, https://doi.org/10.5194/acp-16-10283-2016, 2016.