the Creative Commons Attribution 4.0 License.
the Creative Commons Attribution 4.0 License.
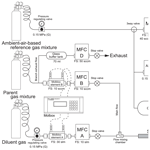
A high-accuracy dynamic dilution method for generating reference gas mixtures of carbonyl sulfide at sub-nanomole-per-mole levels for long-term atmospheric observation
Hideki Nara
Takuya Saito
Taku Umezawa
Yasunori Tohjima
Atmospheric carbonyl sulfide (COS) has received increasing attention as a potential tracer for investigating the global carbon cycle. Owing to the irreversible photosynthetic absorption of COS, changes in the atmospheric COS mole fraction can be related to terrestrial gross primary production. However, the instability of COS in high-pressure cylinders has hampered the accurate determination of atmospheric COS. Here, we report a dynamic dilution method for generating reference gas mixtures containing COS at ambient levels (ca. 500 pmol mol−1). Our method combined a dynamic dilution system employing a high-accuracy mass flow measurement system and a dry reference gas mixture prepared gravimetrically as a parent gas mixture containing a micromole-per-mole level of COS filled in a high-pressure aluminium cylinder. The storage stability of COS at this level was experimentally validated for three gravimetrically prepared dry reference gases over a period of more than 1 decade. We evaluated the dilution performance of the developed method using a gravimetric parent gas mixture containing approximately 1 µmol mol−1 of COS and chlorodifluoromethane (HCFC-22). Excellent repeatability (0.2 % for COS and 0.4 % for HCFC-22 in terms of relative standard deviation; RSD), reproducibility (COS: 0.1 %; HCFC-22: 0.3 %), and dilution linearity (R2>0.99 for both COS and HCFC-22) were obtained and were corroborated by the nearly constant ratio of the normalized gas chromatography–mass spectrometry (GC/MS) response of COS to HCFC-22. The dilution accuracy was examined by comparing the determined HCFC-22 mole fractions in a dynamically diluted parent gas mixture from a mass flow rate measurement system and GC/MS calibrated using a gravimetrically diluted parent gas mixture. The mole fractions of HCFC-22 from these two methods agreed within an acceptable difference of approximately 2 pmol mol−1, validating the dilution accuracy of the developed method. By re-evaluating the experimental data, we determined the mole fractions of COS and HCFC-22 in an ambient-air-based reference gas mixture, with relative standard deviations of 0.1 % for COS and 0.3 % for HCFC-22. These results demonstrated that the developed method can accurately generate reference gas mixtures containing COS at ambient levels, which we expect will support long-term observations of atmospheric COS.
- Article
(2212 KB) - Full-text XML
-
Supplement
(811 KB) - BibTeX
- EndNote
Carbonyl sulfide (COS) is the most abundant sulfur-containing compound in the atmosphere. It acts as a sulfur carrier from the troposphere to the stratosphere, contributing to the distribution of stratospheric sulfate aerosols that influence Earth's radiative balance (Chin and Davis, 1995; Kjellstrom, 1998; Brühl et al., 2012). Atmospheric COS has recently received increasing attention as a potential tracer for examining the global terrestrial carbon cycle (Montzka et al., 2007). Unlike carbon dioxide, COS is irreversibly taken up by terrestrial plants via photosynthesis (Goldan et al., 1988; Protoschill-Krebs et al., 1996; Sandoval-Soto et al., 2005; Seibt et al., 2010; Stimler et al., 2010, 2012), allowing the estimation of terrestrial gross primary production from the local to the global scale based on atmospheric COS observations (Campbell et al., 2008; Blonquist et al., 2011; Asaf et al., 2013; Commane et al., 2013; Maseyk et al., 2014; Wehr et al., 2016; Yang et al., 2018; Kooijmans et al., 2019). For example, the interannual variability in the global annual mean mole fraction of atmospheric COS has been used to estimate the biogeochemical feedback of terrestrial plant ecosystems in response to an increase in atmospheric CO2 levels (Campbell et al., 2017). The World Meteorological Organization has estimated the interannual variability of COS to be only several picomoles per mole in the global background atmosphere over the last 2 decades (WMO, 2018), highlighting the need for technical methods that allow accurate determination of atmospheric COS levels.
Despite promising new directions for estimating global terrestrial gross primary production using COS, atmospheric COS observations are insufficient for understanding its global distribution and budget. One fundamental reason for this is the difficulty in establishing reliable reference gas mixtures containing ambient levels of COS (ca. 500 pmol mol−1). Hall et al. (2014) reported significant differences in the COS measurements from the laboratories from the intercomparison experiment. This might be attributed to the instability of COS stored in high-pressure cylinders. Indeed, as demonstrated in this study (see Fig. 3 in Sect. 3), the changes in the mole fraction of COS dry gas mixture stored in the cylinder during storage can be greater than the interannual variability in COS. To better understand the spatial and temporal variations in atmospheric COS, observations on a stable calibration scale that is compatible across multiple laboratories are required. Thus, approaches that address issues related to the storage instability of COS are required.
Gravimetric preparation, in which individual gas components are weighed in a cylinder, is the most common approach for producing reference gas mixtures containing long-lived trace gases such as CO2, CH4, and N2O (ISO, 2015). Although this approach is the most accurate, it is time-consuming and generally requires multistep dilutions when preparing a gas mixture at the sub-nanomole-per-mole level. This multistep procedure results in the accumulation of preparation uncertainty from weighing, and, in addition, gravimetric dilution in cylinders may introduce uncertainties related to surface effects that can vary by cylinder type.
Dynamic dilution is an alternative approach to gravimetric preparation (ISO, 2018). Historically, various dynamic dilution methods have been applied to gas species that exhibit strong adsorption or limited stability at ambient levels in high-pressure cylinders (Goldan et al., 1986; Fried et al., 1990; Wright et al., 1994; Kerwin et al., 1996; Mohamad et al., 1996; Nakao and Takamoto, 1999?; Goody and Milton, 2002; Brewer et al., 2011, 2014; Flores et al., 2012; Kim et al., 2016; Guillevic et al., 2018; Macé et al., 2022). A major advantage of dynamic dilution is that the reference gas mixture can be generated from a pristine parent gas mixture immediately before analysis, which eliminates the need for storage and therefore prevents any possible mole fraction change that could occur over time. However, only a few studies have used dynamic dilution to generate diluted gas mixtures at the sub-nanomole-per-mole level with high accuracy (Kim et al., 2016; Guillevic et al., 2018). Moreover, the application of this method is limited to gas species with a clear stability at high mole fractions in high-pressure cylinders. To the best of our knowledge, there have been no reports on dynamic dilution-based techniques for generating sub-nanomole-per-mole reference gas mixtures of COS.
Here, we report a dynamic dilution method for the preparation of reference gas mixtures containing COS at ambient levels, which we expect will support long-term atmospheric COS observations. In Sect. 2, we present a detailed description of the dynamic dilution system developed in this study, estimate the theoretical dilution uncertainty, and describe the procedure for determining the mole fraction of COS in a generated gas mixture. In Sect. 3, we examine the long-term stability of a dry reference gas mixture of COS stored in a high-pressure aluminium cylinder. In Sect. 4, we evaluate the dilution performance and overall dilution accuracy of our method and provide an example of the mole fraction assignment for COS in an ambient-air-based reference gas mixture. In Sect. 5, we discuss the possible causes of dilution bias observed for COS in this study. All experiments were designed based on a series of preliminary studies (hereafter referred to as our pilot study), which are detailed in the Supplement.
Figure 1 shows the schematic of our dynamic dilution system. Briefly, the system generates the desired gas mixture by blending two gas flows of a gravimetrically prepared parent gas mixture containing a few micromole-per-mole fractions of COS and a COS-free diluent gas. Dilution accuracy is achieved by a high-accuracy flow measurement system that has been used as a dynamic dilution method to generate sub-micromole-per-mole reference gas mixtures and adjust the composition of gas matrices (Brewer et al., 2014; Nara et al., 2012). A detailed description of the main components of the dilution system is provided in Sect. 2.1, a general explanation of the generation of a dynamically diluted gas mixture is provided in Sect. 2.2, a theoretical estimation of the dilution uncertainty is discussed in Sect. 2.3, and the determination of the COS mole fraction in a dynamically generated gas mixture combined with a gas chromatography–mass spectrometry (GC/MS)-based sample determination system (hereafter referred as GC/MS measurement system) is provided in Sect. 2.4.
2.1 Main components of the dilution system
The dilution system consists of two gas cylinders, a high-accuracy flow measurement system, four mass flow controllers (MFCs), a static mixer, and an automatic back-pressure regulator (ABPR). These components are described in detail below.
2.1.1 Parent and diluent gases
A dry gas mixture of COS and chlorodifluoromethane (HCFC-22) was used as the parent gas, with a high-purity nitrogen balance. The parent gas mixture was prepared gravimetrically by a Japanese gas manufacturer (Japan Fine Products Co., Kanagawa, Japan) and was provided in a Luxfer 9.4 L high-pressure aluminium cylinder which was fitted with a brass-bodied SUS316L metal diaphragm valve (G55-L; Hamai Industries Limited, Tokyo, Japan) at a fill pressure of 9.81 MPa (unless otherwise noted, gas pressures are reported on the gauge scale). The inner surface of the cylinder was polished using a proprietary method to reduce adsorption of COS and HCFC-22. The nominal mole fractions of COS and HCFC-22 were 1.01 and 1.00 µmol mol−1, respectively. HCFC-22 was added to the parent gas mixture to aid in assessing the presence and extent of the dilution bias (see details in the Supplement). HCFC-22 was selected because (1) its atmospheric dry mole fraction is relatively close to that of COS (approximately 260 pmol mol−1 in northern hemispheric background air; cf. COS: 400–600 pmol mol−1) (WMO, 2022) and (2) it is more stable than COS with respect to adsorption. High-purity nitrogen (> 99.99995% purity; Japan Fine Products, Co.) filled in a 47 L high-pressure manganese steel cylinder was used as the diluent. The pressures of the parent and diluent gases supplied from respective cylinders were regulated using single-stage (AEROTRACE; Nissan Tanaka Corp., Saitama, Japan) and two-stage stainless-steel pressure regulators (SuperLabo; Nissan Tanaka Corp.), respectively. The former regulator had an SUS316L body with an SUS316L metal diaphragm, and the internal gas contact surface was SUS316L and fluorine resin.
The presence of COS as a contaminant in the diluent gas was verified before each experiment using a GC/MS measurement system (see Sect. 2.4). Repeated measurements of the diluent gas showed that small amounts of COS (but not HCFC-22) were detected initially, but the amounts decreased with the number of measurements and were finally below the detection limit. This COS decrease was most likely ascribed to adsorption/desorption in the GC/MS system, rather than the contamination from the dilution gas and from the helium gas used as the carrier gas for the GC/MS system. These repeated measurements of the diluent gas ensured that there was no COS contamination from the diluent or carrier gases, which may have biased the determination of COS and HCFC-22 in our experiment.
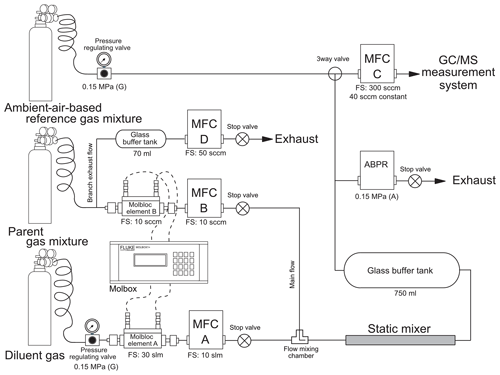
Figure 1Schematic of the dynamic dilution system. The thermal-based mass flow controllers and automatic back-pressure regulator are denoted as MFC and ABPR, respectively. Full-scale volume flow rates (FS) that determine the flow precision are provided for each Molbloc element and thermal-based mass flow controller. The ambient-air-based reference gas mixture was used to calibrate the GC/MS system (see details in Sect. 2.4.).
2.1.2 High-precision flow measurement system
A Molbloc high-precision flow measurement system (Fluke Co., WA) was used to measure the mass flow rates of the diluent and parent gases. The system comprised a system controller (Molbox 1+) and two Molbloc-L laminar mass flow elements (A, model 3E4 VCR-V-Q, and B, model 1E1 VCR-V-Q). The full-scale volume flow rates of the diluent and parent gases were 30 standard litres per minute (slm) and 10 standard cubic centimetres per minute (sccm), respectively. The Molbloc system accurately calculates the mass flow rate based on measurements of the temperature and the upstream and downstream pressures of the gas flow passing through the element in a laminar regime according to the Hagen–Poiseuille law (Landau and Lifshitz, 1987). System control and data acquisition were performed using the Tera Term software (version 4.97; Tera Term, 2017). The maximum measurement uncertainty depended on the flow rate and was ±0.2 % of the measured value or ±0.02 % of the full-scale flow rate at flow rates above and below 10 % of the full-scale flow rate. The gas contact area along the entire flow path of the Molbloc elements was SUS316L.
2.1.3 Thermal-based mass flow controllers
Our dynamic dilution system used four thermal-based MFCs (A–D). MFC-A and -B (SEC-Z712MGX; HORIBA STEC, Co., Ltd., Kyoto, Japan) were used for automatic flow control of the diluent and parent gases, respectively, with full-scale flow rates of 10 slm and 10 sccm, respectively. MFC-C was the same model as the controller A and B but with a full-scale flow rate of 300 sccm and was used to control the outflow of the dynamically generated gas mixture from the dilution system. The flow precision of these three MFCs was ±1.0 % of the set point or ±0.25 % of the full-scale flow rate for above and below 25 % of their full-scale flow rate. For these MFCs, SUS316L and Co–Ni alloys were used as the wetted-part material of which surfaces were mirror-polished to prevent gas adsorption. The fourth controller, MFC-D (SEC-400MK3; HORIBA STEC, Co., Ltd.), was used to control the exhaust of the excess parent gas for dynamic buffering of the parent gas pressure with a precision of 2 % for a full-scale flow rate of 100 sccm, with a limited operating range of over 5 % of the full-scale flow rate. Each MFC was operated individually using a control unit (PD-D20; HORIBA STEC, Co., Ltd.).
2.1.4 Static mixer
A static mixer (G 1/4-18; Noritake, Co., Ltd., Aichi, Japan) was used to facilitate blending of the diluent and parent gas flows. The gas mixer was a 1/4-inch internal diameter SUS316LS stainless-steel pipe with a length of 215 mm. The mixing fin assembly consisted of 18 elements and was set within the pipe. The inner surface of the pipe and outer surfaces of the mixing fin assembly were electrically polished to minimize gas adsorption.
2.1.5 Automatic back-pressure regulator
An ABPR (UR-Z722M-UC-B; HORIBA STEC, Co., Ltd.) was used to exhaust the excess diluted gas mixture from the system and ensure that the pressure within the system remained constant. The ABPR operated at an absolute operating pressure range of 10–300 kPa and controlled the pressure with a precision of 0.5 % for the full-scale operating pressure (corresponding to ±1500 Pa), resulting in the pressure inside the system being independent of the barometric variations. The ABPR was controlled using the same unit as that used to control the MFCs. The gas contact area along the entire flow path was an all-metal construction made of SUS316L with a mirror-polished surface.
2.2 General description of the dynamic dilution system
Our dynamic dilution system is similar to that developed by Brewer et al. (2014) but was modified by installing a branch exhaust system to accurately generate gas mixtures containing sub-nanomolar-per-mole levels of COS.
When starting the system, we purged it for at least 15 min with the parent and diluent gases supplied from the high-pressure cylinders at the flow rates set in the subsequent dilution experiment. Before each gas dilution, a conditioning purge was performed for 15 min (only the first gas dilution needed at least a 30 min purge). During this 15 min purge, the pressures of the two gases' downstream respective Molbloc elements were adjusted to match each other within ±0.3 kPa based on the pressure readings from the Molbloc elements. Although the mass flow rates of the two gas flows were controlled by MFC-A and -B, using these MFCs alone resulted in insufficient pressure-matching between the two flows. Therefore, for more precise pressure control, additional pressure stabilization techniques were used for each gas flow because their mass flow rates were substantially different; for example, the flow rate of the diluent gas was 2000 times larger than that of the parent gas when generating a diluted gas mixture containing COS at ambient levels of approximately 500 pmol mol−1.
The pressure of the diluent gas was controlled using a cylindrical regulator and a simple in-line pressure regulator (6600A; Kofloc Corp., Kyoto, Japan). The pressure of the diluent gas was first set at 0.24 MPa using the cylinder regulator and was further adjusted using the in-line pressure regulator set at 0.15 MPa to suppress the pressure change in response to regulator cooling owing to adiabatic expansion of the outflowing gas. In contrast, the parent gas was introduced into the flow-mixing chamber along the main flow path at a flow rate usually limited to 10 sccm, with the pressure set by the cylinder regulator at 0.15 MPa. At such a low flow rate, the instability of the cylinder regulator becomes pronounced, leading to pressure fluctuations of several thousand pascals. Because this pressure fluctuation is difficult to remove by passing through an MFC, we installed a branch exhaust system located upstream of the flow-measuring element in the Molbloc system. The system exhausted the parent gas along the branch exhaust flow path via a 70 mL glass buffer tank at a flow rate of more than 10 sccm, which was higher than the flow rate of the main flow. This branch exhaust system prevented the dilution biases found in our pilot study (see Supplement: the dilution biases are discussed in detail in Sect. 5) and dynamically buffered any pressure changes to facilitate the stabilization of the parent gas pressure, which should match that of the diluent gas during gas dilution. The diluent and parent gas flows were controlled by MFC-A and -B, which were placed in the respective flow paths downstream of the Molbloc elements and converged in a flow-mixing chamber. The flow-mixing chamber was made of a stainless-steel T-fitting of 1/4-inch outer diameter, in which one port was connected to a bored-through reducer. The diluent gas flowed straight from upstream to downstream, while the parent gas tube (1/16-inch outer diameter) was inserted from the side port of the T-fitting through the reducer and was bent at a right angle in the downstream direction so that the parent gas flow was introduced into the diluent gas flow in the flow direction. After the convergence of the two flows in the chamber, the generated gas mixture was passed through the static mixer to complete gas blending and then into a glass tank (volume: 750 mL) to buffer any pressure changes in the blended gas mixture.
Finally, the diluted gas mixture was supplied to the GC/MS measurement system via MFC-C. Excess flow was exhausted through the ABPR set at an absolute back-pressure of 0.15 MPa, which prevented the influence of barometric changes outside the system. The expected mole fractions of COS and HCFC-22 in the generated gas mixture were accurately calculated from the mass flow rates measured using the Molbloc system. To prevent the possible loss of COS by adsorption, a Sulfinert coating (Restek Corporation, PA, USA) was applied to all tubes, fittings, and valves that were exposed to the parent gas and generated gas mixtures, except the static mixer.
2.3 Estimation of uncertainty for the gas dilution
The mole fraction of gas x in a dynamically generated gas mixture can be calculated using the mole fraction of the target gas in the gravimetric parent gas mixture, mass flow rates of the parent and diluent gases under the assumption of complete mixing of the two gases, and constant mole fraction of gas x in the gas flow from the parent gas cylinder. The mole fraction of gas x is expressed as follows:
where [x] indicates the mole fraction of gas x in the gas flow of the dynamically generated gas mixture (diluted) and parent gas (parent) and f is the mass flow rate corresponding to the subscripted gas flow. By applying the propagation law to this equation, the uncertainty (udiluted) in the mole fraction of the diluted gas x can be estimated from the uncertainty of each variable associated with the gravimetric preparation and Molbloc measurement as follows:
where each δ symbol represents the uncertainty of corresponding variables [x]parent, fparent and fdiluent. The dilution uncertainty associated only with the Molbloc measurements can be estimated by removing the uncertainty derived from the gravimetric preparation of the parent gas (δ[x]parent=0). The mole fraction distributions of gas x and relevant dilution uncertainties expressed as a relative standard deviation (RSD) are shown in Fig. 2. The dilution system can generate diluted gas mixtures across a wide range of mole fractions depending on the flow rates of the diluent and parent gases, covering the typical mole fractions of COS in the atmosphere (400–600 pmol mol−1). The dilution uncertainty was determined using the flow rates and was found to change significantly at flow rates corresponding to 10 % full-scale for the Molbloc elements used. For example, within the typical atmospheric COS mole fraction range, the uncertainty at parent gas flow rates below 1 sccm, which corresponds to a 10 % full-scale flow rate, increased rapidly from approximately 0.5 % with a decreasing flow rate. In contrast, the uncertainty remained nearly constant at parent gas flow rates above 1 sccm. The parent gas flow rates above 2 sccm within the typical COS mole fraction range suppressed the dilution uncertainty to below 0.3 %.
2.4 Determination of COS in a diluted gas mixture
To evaluate the dilution performance of our method, we measured the mole fractions of COS and HCFC-22 (hereafter “target gases”) in dynamically diluted gas mixtures by using the GC/MS measurement system. Because the method used for target gas determination including the basic configuration of the measurement system was presented in Saito et al. (2010), only a brief description is provided here. The gas mixture generated by the dilution system was introduced via MFC-C into the GC/MS measurement system for dual-stage preconcentration of target gases. The gas mixture passed through a preconcentration trap filled with HayeSep D cooled below −130 °C at a constant flow rate of 40 sccm for 12 min, while the mass flow rates of the parent and diluent gases were recorded to calculate the mole fractions of the target gases. The preconcentration trap was heated to −70 °C to release major atmospheric constituents and then up to 100 °C to transfer the trapped gases to a cryofocusing trap filled with HayeSep D cooled at −85 °C. The trap was then heated to 100 °C to inject the trapped gases onto a PoraBOND Q separation column for subsequent MS analysis. The total measurement time for each sample was 1 h.
The target gas measurements were referenced against measurements of an ambient-air-based reference gas mixture to correct for diurnal variations in the sensitivity of the mass spectrometer. To prepare the reference gas mixture, ambient air was dried to a dew-point temperature of less than −80 °C by passing it through a Nafion Perma Pure dryer (Perma Pure LLC; Toms River, NJ, USA) and a chemical trap packed with phosphorous pentoxide (Sicapent®; EMD Millipore, Billerica, MA, USA) and was subsequently filled into a 48 L high-pressure aluminium cylinder (Luxfer) fitted with a brass-bodied cylinder valve (G55-L; Hamai Industries Limited). The inner surface of the cylinder was polished and anodized using a proprietary method (Japan Fine Products, Co.). Before filling, the aluminium cylinder was evacuated, and Milli-Q water was added so that the water vapour mole fraction was approximately 500 µmol mol−1 at a maximum fill pressure of 14.7 MPa after gas filling to enhance the stability of the trace gases in the compressed air during storage (Montzka et al., 2004; Hall et al., 2014).
The target gases in the reference gas mixture were measured before and after sample gas measurement. The signal responses obtained from the GC/MS measurements were used to estimate the change in GC/MS sensitivity during sample measurements by linear interpolation. The signals from the sample measurements were normalized to the interpolated responses of the reference gas mixture and then multiplied by the known mole fraction of the corresponding target gas in the reference gas mixture as follows:
where the mole fractions of gas x in the sample and the reference gas mixture are indicated in square brackets and Rx,sample and are the signal responses for gas x in the sample gas mixture and the interpolated response for the reference gas mixture, respectively. The typical repeatability for COS and HCFC-22 determined from repeated measurement of a compressed cylinder gas was an RSD of approximately 0.5 % during the study period.
Atmospheric trace gases with dry mole fractions of sub-nanomole-per-mole levels, such as COS and halocarbons, can exhibit significant changes during storage in high-pressure cylinders. High-pressure stainless-steel cylinders are generally used for the storage of reference gas mixtures for these trace gases. Previous studies have reported that COS and halocarbons show superior stability, even at ambient mole fractions, in this type of cylinder (Hall et al., 2014; Guillevic et al., 2018). Thus, the use of stainless-steel cylinders can be an effective measure to reduce changes in the mole fractions of COS during storage. However, in this study, we opted to use Luxfer 9.4 L aluminium cylinders with a brass-bodied cylinder valve (G55-L; Hamai Industries Limited) because aluminium cylinders with treated inner surfaces are easier for us to obtain than stainless-steel cylinders.
In the past, we prepared several dry reference gas mixtures of COS at ambient levels filled in high-pressure aluminium cylinders by the gravimetric method, and almost all of them showed substantial changes in the mole fraction of COS during storage, which is hereafter referred to as “drift”. Figure 3 shows an example of drift, in which we observed a substantial decrease in the mole fraction of COS. Although the stability during storage could be enhanced depending on the material of the gas cylinder and inner-surface treatment (Yokohata et al., 1985; Montzka et al., 2004, 2007; Hall et al., 2014), other factors that affect the amount and rate of this drift remain unknown.
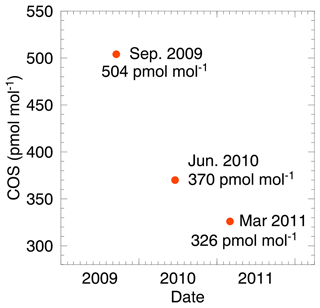
Figure 3Time series of COS mole fraction in a non-passivated high-pressure aluminium cylinder. The standard gas was prepared gravimetrically to contain approximately 500 pmol mol−1 COS in dry nitrogen.
At a micromole-per-mole level of COS, we found no notable COS drift during storage in high-pressure aluminium cylinders from a stability check experiment. In the experiment, compressed natural air was measured using the GC/MS measurement system against three different dry reference gas mixtures containing approximately 5 µmol mol−1 of COS prepared in different years. These reference gas mixtures (balanced in nitrogen) were prepared gravimetrically and filled into Luxfer 9.4 L aluminium cylinders with the brass-bodied cylinder valves at a fill pressure of 9.81 MPa by a Japanese manufacturer (Japan Fine Products, Co.) in 2006, 2011, and 2015. The nominal mole fractions were 5.12, 5.02, and 5.05 µmol mol−1, respectively (Table 1).
For the determination of COS, we used an established automated measurement system combining a commercially available dynamic diluter (SGGU-7000NS-U6; HORIBA STEC, Co., Ltd.) and the GC/MS measurement system. The GC/MS measurements of the compressed natural air were referenced to those of a gas mixture containing approximately 500 pmol mol−1 of COS, which was dynamically diluted 10 000 times with high-purity nitrogen (> 99.99995 % purity) from the gravimetrically prepared reference gas mixture using the commercial diluter. We do not present the details of the diluter here; only the general features are described. The diluter employed a two-step dilution method, and the dilution was performed by mixing the parent and diluent gases at gas flow rates regulated by two MFCs. The expected mole fraction from dilution was calculated from the measured flow rates of the two gases. The nominal precision of the dilutions was ± 1.0 %. The COS determination by GC/MS was repeated four to eight times according to the protocol described in Sect. 2.4, until high repeatability was obtained in the measured values. The COS mole fraction was determined based on the final three measurements.
As presented in Table 1, the determined mole fractions of COS can be compared with each other to indirectly evaluate the long-term stability of COS at the micromole-per-mole level in the reference gas mixtures during storage. The mole fractions of COS from each experiment corresponding to different parent gas mixtures prepared in different years were determined with standard deviations (1σ) of less than 0.5 pmol mol−1. While the individual average COS values did not agree within the 2-σ envelope of their corresponding measurement data, they were within 2 % of the average values. It is difficult to clarify whether a marked COS drift occurred in the reference gas mixture owing to the unquantified combined uncertainty related to gravimetric preparation and dynamic dilution. However, the observed relationship presented in Table 1 indicates that the nominal COS values provided by the gas manufacturer at the time of preparation were almost consistent for all three cylinders, at least at the time of this experiment. These results imply that in our experiments the impact of drift during storage is practically negligible over a period of more than 1 decade when using a gas mixture containing COS at a micromole-per-mole level. This suggests an alternative approach to maintaining the COS calibration scale with a high-accuracy dynamic dilution of the quasi-stable reference gas mixture. Although periodic absolute determination is still required for stability validation, its frequency can be reduced significantly.
Note that we used the automated measurement system using the commercial diluter, which was convenient for detecting significant levels of COS drift, whereas the dynamic dilution system using the Molbloc system developed in this study required manual dynamic gas dilution and subsequent GC/MS measurements. However, the commercial diluter needs more time for system conditioning and has an inferior dilution accuracy to the developed dilution system. Therefore, the dynamic dilution system using the Molbloc system offers significant advantages for the accurate determination of COS in a reference gas mixture.
We investigated the dilution performance of the developed dynamic dilution system in terms of precision and accuracy, comprised of repeatability and reproducibility and of trueness and precision, respectively (ISO, 2017). The linearity of the developed dynamic dilution system was also investigated. These investigations were conducted based on GC/MS analysis of the target gases, and we report the GC/MS measurements as response values normalized to an ambient-air-based reference gas mixture according to the procedure described in Sect. 2.4. After validating our dilution method, we assigned mole fractions to the target gases in the ambient-air-based reference gas mixture.
4.1 Repeatability and reproducibility
To evaluate the dilution precision, which consists of repeatability and reproducibility, we conducted a series of experiments in which we generated a diluted parent gas mixture using a dilution system, followed by GC/MS measurements of the target gases. A total of five experiments were conducted, with each experiment consisting of at least four sample measurements. Four of the experiments were performed on different days within a week, and the final experiment was performed approximately two weeks after the previous four experiments. A common dilution ratio was used in these experiments. The expected mole fraction of the diluted target gases was approximately 500 pmol mol−1 for COS and 495 pmol mol−1 for HCFC-22, and the flow rates of the parent and diluent gases to be blended were set at 2.5 and 5000 sccm, respectively. For each experiment, the average and standard deviation of the response values from the GC/MS measurements were calculated from at least four normalized responses.
Figure 4 shows the results of the GC/MS measurements of the diluted target gases. Dilution repeatability was calculated as the RSD of the normalized responses obtained in each experiment. The obtained RSD values for COS and HCFC-22 from each of the five experiments were within 0.3 % and 0.6 %, respectively, and the average RSD values were 0.2 % and 0.4 %, respectively. Although these RSD values of the dilution repeatability were determined as the combined uncertainties from the GC/MS measurements and the dynamic dilution, the average RSD values were comparable with those of the typical measurement repeatability of the GC/MS system (approximately 0.5 %). These results suggest that the dilution repeatability obtained was primarily subject to the GC/MS measurements rather than from the dynamic dilution. Conversely, the dilution reproducibility can be evaluated through the standard deviation of the average normalized responses obtained from each experiment. The averages for both COS and HCFC-22 from the five experiments were in good agreement. The RSD values for these responses were 0.1 % and 0.3 % for COS and HCFC-22, respectively, which were reduced compared with those from the repeatability test. These results suggest that the uncertainty in target gas measurements was dominated by random uncertainty from the GC/MS measurements and that there was no significant systematic uncertainty observed between the experiments. This is also corroborated by the ratio of the normalized response of COS to that of HCFC-22. We observed no clear systematic changes in the normalized response ratios in each experiment and obtained nearly constant average ratios among the five experiments. Based on the evaluation of the repeatability and reproducibility, the dilution system was found to generate a dynamically diluted gas mixture precisely, which can be represented by the repeatability of the GC/MS measurements.
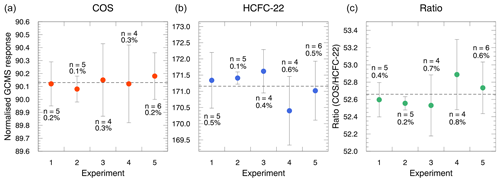
Figure 4Repeatability and reproducibility of our dynamic dilution method for generating gas mixtures containing COS and HCFC-22. Vertical error bars indicate the standard deviation of the normalized values obtained in each experiment. The number of measurements and relative standard deviation are shown for each average plot.
4.2 Dilution linearity
The dilution linearity was examined by comparing the determined target gases in the generated gas mixture by the Molbloc system with those obtained by the GC/MS measurement system. The mole fractions of the target gases were calculated from the mass flow rates of the parent and diluent gases measured using the Molbloc system, whereas the target gases were determined as normalized GC/MS responses based on GC/MS measurements. For the experiment, the dilution ratio was varied by changing the parent gas flow rate using MFC-B such that the expected mole fraction of COS in the generated gas mixture was in the range of 400–600 pmol mol−1, which corresponds to the typical atmospheric mole fraction. We checked the linear response of COS and HCFC-22 in increments of 50 pmol mol−1 in the range defined by the dilution ratio, and then we checked it in decrements of 50 pmol mol−1 to investigate the possible memory effect of the previous dilution process. The normalized GC/MS responses were plotted against the mole fractions obtained from the Molbloc system (Fig. 5). For the increment and decrement changes, the normalized COS and HCFC-22 values showed excellent linearity with the corresponding mole fractions from the Molbloc system, and linear least-squares fitting yielded determination coefficients greater than 0.99. The relative residuals of the normalized values from the fitting line were also plotted (bottom panels). The values for COS and HCFC-22 were distributed within 0.4 %, which can be explained by the typical measurement repeatability (approximately 0.5 %) of the GC/MS measurement system. There was no clear dependence of the relative residual values on the Molbloc mole fraction, and similar results were obtained for the ratio of the normalized values (data not shown). These results indicated that there was no significant memory effect from the previous blending process. Overall, our results demonstrate that our dynamic dilution system has excellent linearity in the range of the investigated dilution ratios and shows no bias depending on the mole fraction of the generated target gas.
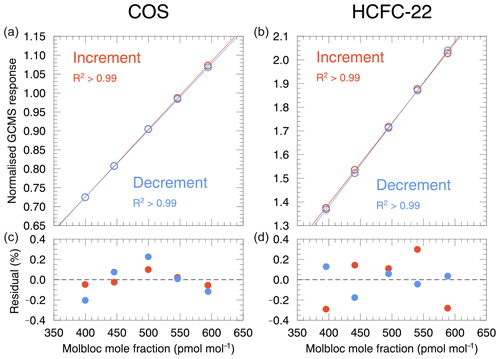
Figure 5Dilution linearity of the dynamic dilution method for COS and HCFC-22 in response to increases or decreases in the generated target gas mole fractions. The normalized GC/MS responses (a, b) and residuals from the fitting line determined by linear least-squares (c, d) are shown as a function of the mole fraction calculated from the average mass flow rates during the sample concentration process measured by the Molbloc system.
4.3 Validation of dilution accuracy
Subsequently, the dilution accuracy, comprising trueness and precision, of the dynamic dilution method was assessed using a validation experiment. In this experiment, the parent gas mixture was dynamically diluted using the developed dynamic dilution system, and the resulting diluted parent gas mixture was analysed through GC/MS calibrated with the gravimetrically diluted parent gas mixture. The determined mole fractions of HCFC-22 from the GC/MS measurements were compared with those from the Molbloc system based on the measurements of the mass flow rates of the parent gas mixture and diluent gas (Fig. 6). The diluted parent gas mixture for GC/MS calibration was prepared by a Japanese gas manufacturer (Japan Fine Products, Co.) by the gravimetric dilution of the parent gas mixture with high-purity nitrogen (> 99.99995 % purity). This mixture was filled at a fill pressure of 9.4 MPa into a Luxfer 9.4 L high-pressure aluminium cylinder fitted with a brass-bodied SUS316L metal diaphragm valve (G55-L; Hamai Industries Limited). The inner surface of the cylinder was polished using a proprietary method (Japan Fine Products, Co.). The mole fractions of COS and HCFC-22 in the gas mixture were 505.0 and 500.4 pmol mol−1, respectively. Owing to this gravimetric dilution, the GC/MS measurements were standardized to those of the parent gas mixture. We expected a significant loss of COS in this gravimetric gas preparation process, and a marked decrease in COS was observed: the nominal value reduced to approximately 1/13 after preparation of the reference gas mixture, probably within 1 month. However, we could analyse the dilution accuracy based on the determination of HCFC-22 as our experiments demonstrated comparable dilution performances between HCFC-22 and COS, with no significant systematic dilution bias for COS, as presented in the Supplement.
The dynamically diluted parent gas mixture was generated by a 2020-fold dilution to produce an expected HCFC-22 mole fraction of approximately 495 pmol mol−1. The generated gas mixture was measured six times in series by GC/MS, and the average HCFC-22 mole fraction in the diluted gas mixture during the preconcentration process of the GC/MS measurement was determined using the Molbloc system (Table 2). We obtained average HCFC-22 values with high precision using both methods. The standard deviations for the GC/MS and Molbloc measurements were 0.8 and 0.1 pmol mol−1, and the corresponding RSD values were 0.2 % and 0.1 %, respectively. There was a slight difference between the two average values: the GC/MS measurements were approximately 2 pmol mol−1 lower than the Molbloc measurements. The average values fell outside the 2-σ range of one another, indicating a potential difference in their trueness.
Based on the mass flow rates measured by the Molbloc system, we calculated the average HCFC-22 mole fraction in the diluted gas mixture during the preconcentration process of the GC/MS measurements, which was directly traceable to the nominal HCFC-22 value contained in the parent gas mixture. Conversely, the GC/MS was calibrated with the gravimetrically diluted parent gas mixture with traceability to the parent gas mixture to determine the HCFC-22 value. However, this method includes the uncertainty derived from the gravimetric dilution. Although no estimated uncertainty was provided by the manufacturer, the preparation uncertainty can be roughly estimated based on the typical measurement uncertainty of a weighing scale (± 25 mg, 2σ) and was ± 1.2 pmol mol−1 for HCFC-22. Since this uncertainty is propagated to the GC/MS measurement, the observed difference of approximately 2 pmol mol−1 was acceptable within the expanded uncertainty of 2.8 pmol mol−1 (k=2) for the GC/MS measurements calculated from the combined uncertainty of the GC/MS measurements and gravimetric preparation. Based on these results, we concluded that the dynamic dilution method could accurately generate COS reference gas mixtures at ambient levels from those at micromole-per-mole levels.
4.4 Mole fraction assignment of COS and HCFC-22 in a high-pressure aluminium cylinder
Here, we present an example of mole fraction assignment for target gases in a high-pressure aluminium cylinder using dynamic dilution and the GC/MS measurement systems. By re-evaluating the experimental data described in Sect. 4.1, we determined the mole fractions of COS and HCFC-22 in the ambient-air-based reference gas mixture, which was used to correct for diurnal variations in the sensitivity of GC/MS. As described in Sect. 2.4, the mole fraction of gas x was expressed as the product of the normalized GC/MS response and the assigned mole fraction of gas x in a reference gas mixture according to Eq. (3). Rearranging Eq. (3) using the mole fraction determined from the Molbloc measurements yields
where Rx,diluted is the normalized GC/MS response for gas x in the dynamically diluted gas mixture and [x]diluted,Molbloc is the average mole fraction calculated from the mass flow rates of the parent and diluent gases measured by the Molbloc system during sample concentration. The assigned mole fraction values for the target gases in the ambient-air-based reference gas mixture are summarized in Table 3. The assigned values of COS and HCFC-22 were 554.5±0.1 and 289.1±0.3 pmol mol−1, respectively. These results suggest that the determination of COS and HCFC-22 contained in an ambient-air-based reference gas mixture used to calibrate the GC/MS system was performed with an accuracy that enables the analysis of the spatiotemporal variability of the target gases – in particular, the interannual variability in COS. However, the obtained values were determined against a gravimetrically prepared parent gas mixture. Therefore, the absolute determination of the target gases in the parent gas mixture is required to establish our COS calibration scale for atmospheric COS observations. The major advantage of the developed dynamic dilution method is that COS values determined by our dynamic dilution method can be recalculated on the updated COS calibration scale after the absolute determination, and on the scale from other laboratories through an intercomparison experiment, as long as COS in the parent gas mixture is stable.
Table 3Mole fraction assignments of COS and HCFC-22 contained in an ambient-air-based reference gas mixture based on GC/MS analysis, calibrated with a Molbloc dynamic dilution system using a parent gas mixture containing 1.01 and 1.00 µmol mol−1 of COS and HCFC-22, respectively.
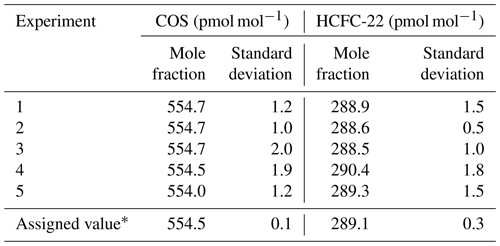
∗ Uncertainty is given as the standard deviation of the determined mole fractions from five sets of experimental data.
We developed a dynamic dilution method for preparing reference gas mixtures containing COS that includes a relative drift-reduction approach to avoid issues related to the change in the COS mole fraction over time; however, outstanding issues still exist that may lead to reduced consumption of the parent gas mixture in our dynamic dilution method.
In our pilot study, we found an unexplained dilution bias responsible for the inconsistent determination of the target gases in the dynamically generated gas mixture between the GC/MS and Molbloc measurements (see Supplement). The dilution bias depended on the pressure and flow rates of the parent and diluent gases; even under pressure control of the relevant gas flows, a greater bias was observed for COS than HCFC-22. We attributed this COS-specific dilution bias to factors possibly associated with the adsorption of COS onto the inner surface of the dilution system or with gas fractionation processes occurring at the cylinder and regulator during the passage of the parent gas flow through them. Therefore, we improved our dilution method by increasing the system purge time, introducing a more rigorous pressure-matching between the two gas flows and increasing the parent gas flow rate beyond that required for generation of reference gas mixture, with excess flow exhausted via a branch exhaust system. Consequently, the combination of these strategies worked well for high-accuracy dynamic dilution in this study, although COS-specific bias is not clearly understood.
A similar dilution bias was observed by Brewer et al. (2014). They reported that the dynamically generated mole fractions of SO2 and CO showed a systematic bias that was inversely proportional to the parent gas flow rate at flow rates of less than 10 mL min−1. In our experiments, the dilution accuracy deteriorated at these flow rates before improvements were made to the system; however, no clear inverse dependence was observed, implying that the dilution bias was not limited to COS dilution and that its impact and biasing can vary in a complicated manner, probably depending on the experimental conditions and physical properties of the diluted gases, especially at a lower flow rate of the parent gas. On the other hand, previous studies identified several factors that can cause gas fractionation in gases flowing from a high-pressure cylinder via a pressure regulator. Gas fractionation can be caused by kinetic processes owing to diffusive fractionation, such as pressure diffusion, thermal diffusion, effusion, and gas adsorption to the inner wall of the cylinder (Langenfelds et al., 2005; Schibig et al., 2018; Hall et al., 2019; Aoki et al., 2022). Given that we previously suggested the possible involvement of the cylinder and regulator, these diffusive processes may contribute to the observed dilution bias, with a strong influence on COS dilution. Further investigations are required to identify the mechanisms underlying the observed dilution bias.
It is important to note that the precision of the developed dynamic dilution method is inferior to that of gravimetry at this stage; however, the developed method has a practical advantage for atmospheric COS observation because of the limited storage stability of COS in high-pressure cylinders. Another advantage is that the new technical knowledge obtained from this study will enable us to establish an automatic dynamic dilution system that can generate a diluted reference gas mixture with precise pressure control of the parent and diluent gases linked to the GC/MS system. This will expand the application of the dilution system to other analytical systems for different target gases and reduce the labour and time required to conduct experiments. For example, the international WMO Global Atmosphere Watch Programme set a goal of ±2 nmol mol−1 for interlaboratory compatibility for atmospheric CO measurements (WMO, 2020). In high-pressure aluminium cylinders, CO is generally known to show substantial positive growth (Novelli et al., 1994, 2003; Tanimoto et al., 2007; Nara et al., 2011), but the impact of CO growth can be relatively reduced at the micromole-per-mole level (Nara et al., 2011), suggesting that our relative drift-reduction approach will most likely maintain the quasi-integrity of CO in the reference gas mixtures at higher CO mole fractions. Because the dilution accuracy of our developed method was estimated to be within 0.1 % RSD for the mole fraction of the generated gas, our dynamic dilution approach can contribute greatly to fulfilling the WMO compatibility goal for CO, even without gravimetric preparation. This emphasizes the usefulness of our dynamic dilution method with a relative drift-reduction approach for maintaining the reference gas scale and traceability of unstable trace gases at ambient levels.
We developed a one-step dynamic dilution method that is capable of accurately generating COS reference gas mixtures at ambient levels (ca. 500 pmol mol−1) from a gravimetrically prepared parent gas mixture containing a high mole fraction of COS (micromole-per-mole level).
We began by investigating the stability of a dry reference gas mixture containing ambient levels of COS stored in a high-pressure aluminium cylinder. Although a substantial decrease in the mole fraction of COS was observed over time, at higher mole fractions (approximately 5 µmol mol−1), COS dry gas mixtures were practically stable over the period of more than 1 decade. These results suggest that the impact of COS drift during storage can be reduced to a negligible level using a gas mixture containing a high mole fraction of COS. By applying this drift-reduction approach, we established a dynamic dilution method that uses a gravimetric parent gas mixture containing approximately 1 µmol mol−1 of COS, together with HCFC-22, which was added to aid in diagnosing the cause of the dilution bias of COS observed in our pilot study.
In the pilot study, the GC/MS response ratio of COS to HCFC-22 revealed an unexplained marked bias for the dilution of the gravimetric parent gas mixture, with a greater bias for COS than for HCFC-22. Although we could not identify the reason for this COS-specific dilution bias, it was considerably improved by implementing strategies against the possible dilution-biasing factors of adsorption and gas fractionation at the cylinder and regulator. The addition of a branch exhaust system was part of this strategy, which helped rinse the flow path in the dilution system and stabilize the pressure of the gravimetric parent gas while exhausting the excess parent gas flow. After implementing these improvements, excellent repeatability and reproducibility were obtained for the dilution of COS and HCFC-22 and for dilution linearity in our evaluation experiments. RSD values of 0.2 % and 0.4 % for COS and 0.1 % and 0.3 % for HCFC-22 were obtained for the repeatability and reproducibility of the method. This excellent dilution performance was corroborated by the nearly constant ratio of the normalized GC/MS response of COS to that of HCFC-22. We obtained a comparable dilution performance between COS and HCFC-22, allowing us to validate the dilution accuracy at ambient levels for HCFC-22 by comparing the flow-rate-based determination using the Molbloc system and the GC/MS-based determination system using a gravimetrically diluted parent gas mixture. A good agreement was obtained between the two methods, demonstrating that our dynamic dilution method can accurately generate diluted gas mixtures without significant systematic bias. Finally, we determined the mole fractions of COS and HCFC-22 in an ambient-air-based reference gas mixture by re-evaluating the experimental data obtained by testing the repeatability and reproducibility of the dilution method. The mole fractions of COS and HCFC-22 were precisely determined, with relative standard deviations of 0.1 % and 0.3 %, respectively.
Overall, these results demonstrated that our dynamic dilution method can contribute to accurate long-term observations of atmospheric COS. However, the absolute determination of COS in a gravimetric parent gas mixture is needed to ensure traceability and interlaboratory compatibility; thus, further development is needed to better understand atmospheric COS dynamics. Notably, our dilution method can be applied to the preparation of other reference gas mixtures containing unstable atmospheric trace gases such as CO in high-pressure cylinders, which emphasizes the usefulness of our dynamic dilution method.
The experimental data related to this article are available upon request from the corresponding author.
The supplement related to this article is available online at: https://doi.org/10.5194/amt-17-5187-2024-supplement.
HN was responsible for the conceptualization, funding acquisition, investigation, and methodology. TS, TU, and YT contributed to the methodology and resources. HN drafted the paper with inputs from TS, TU, and YT. All authors contributed to the discussion and improvement of the paper.
The contact author has declared that none of the authors has any competing interests.
Publisher’s note: Copernicus Publications remains neutral with regard to jurisdictional claims made in the text, published maps, institutional affiliations, or any other geographical representation in this paper. While Copernicus Publications makes every effort to include appropriate place names, the final responsibility lies with the authors.
The authors are grateful to the three anonymous referees for their insightful comments and suggestions. We wish to thank Kentarou Ooyama for his valuable comments on the pressure regulation of gas flow from a high-pressure cylinder. We also thank Nobuyuki Aoki for his valuable comments and technical support in the gravimetric preparation of COS standard gas.
This research was supported by the Japan Society for the Promotion of Science (grant no. 22H05006).
This paper was edited by Huilin Chen and reviewed by three anonymous referees.
Aoki, N., Ishidoya, S., Murayama, S., and Matsumoto, N.: Influence of CO2 adsorption on cylinders and fractionation of CO2 and air during the preparation of a standard mixture, Atmos. Meas. Tech., 15, 5969–5983, https://doi.org/10.5194/amt-15-5969-2022, 2022.
Asaf, D., Rotenberg, E., Tatarinov, F., Dicken, U., Montzka, S. A., and Yakir, D.: Ecosystem photosynthesis inferred from measurements of carbonyl sulphide flux, Nat. Geosci., 6, 186–190, https://doi.org/10.1038/NGEO1730, 2013.
Blonquist Jr., J. M., Montzka, S. A., Munger, J. W., Yakir, D., Desai, A. R., Dragoni, D., Griffis, T. J., Monson, R. K., Scott, R. L., and Bowling, D. R.: The potential of carbonyl sulfide as a proxy for gross primary production at flux tower sites, J. Geophys. Res., 116, G04019, https://doi.org/10.1029/2011JG001723, 2011.
Brewer, P. J., Goody, B. A., Woods, P. T., and Milton, M. J. T.: A dynamic gravimetric standard for trace water, Rev. Sci. Inst., 82, 105102, https://doi.org/10.1063/1.3642660, 2011.
Brewer, P. J., Miñarro, M. D., Di Meane, E. A., and Brown, R. J. C.: A high accuracy dilution system for generating low concentration reference standards of reactive gases, Measurement, 47, 607–612, https://doi.org/10.1016/j.measurement.2013.09.045, 2014.
Brühl, C., Lelieveld, J., Crutzen, P.J., and Tost, H.: The role of carbonyl sulphide as a source of stratospheric sulphate aerosol and its impact on climate, Atmos. Chem. Phys., 12, 1239–1253. https://doi.org/10.5194/acp-12-1239-2012, 2012.
Campbell, J. E., Carmichael, G. R., Chai, T., Mena-Carrasco, M., Tang, Y., Blake, D. R., Blake, N. J., Vay, S. A., Collatz, G. J., Baker, I., Berry, J. A., Montzka, S. A., Sweeney, C., Schnoor, J. L., and Stanier, C. O.: Photosynthetic control of atmospheric carbonyl sulfide during the growing season, Science, 322, 1085–1088, https://doi.org/10.1126/science.1164015, 2008.
Campbell, J. E., Berry, J. A., Seibt, U., Smith, S. J., Montzka, S. A., Launois, T., Belviso, S., Bopp, L., and Laine, M.: Large historical growth in global terrestrial gross primary production, Nature, 544, 84–87, https://doi.org/10.1038/nature22030, 2017.
Chin, M. and Davis, D. D.: A reanalysis of carbonyl sulfide as a source of stratospheric background sulfur aerosol, J. Geophys. Res., 100, 8993–9005, https://doi.org/10.1029/95JD00275, 1995.
Commane, R., Herndon, S. C., Zahniser, M. S., Lerner, B. M., McManus, J. B., Munger, J. W., Nelson, D. D., and Wofsy, S. C.: Carbonyl sulfide in the planetary boundary layer: Coastal and continental influences, J. Geophys. Res., 118, 8001–8009, https://doi.org/10.1002/jgrd.50581, 2013.
Flores, E., Viallon, J., Moussay, P., Idrees, F., and Wielgosz, R. I.: Highly accurate nitrogen dioxide (NO2) in nitrogen standards based on permeation, Anal. Chem., 84, 10283–10290, https://doi.org/10.1021/ac3024153, 2012.
Fried, A., Nunnermacker, L., Cadoff, B., Sams, R., Yates, N., Dorko, W., Dickerson, R., and Winstead, E.: Reference NO2 calibration system for ground-based intercomparisons during NASA's GTE/CITE 2 Mission, J. Geophys. Res., 95, 10139–10146, https://doi.org/10.1029/JD095iD07p10139, 1990.
Goldan, P. D., Kuster, W. C., and Albritton, D. L.: A dynamic dilution system for the production of sub-ppb concentrations of reactive and labile species, Atmos. Environ., 20, 1203–1209, https://doi.org/10.1016/0004-6981(86)90154-X, 1986.
Goldan, P. D., Fall, R., Kuster, W., and Fehsenfeld, F. C.: Uptake of COS by growing vegetation: a major tropospheric sink, J. Geophys. Res., 93, 14186–14192, https://doi.org/10.1029/JD093iD11p1418, 1988.
Goody, B. A. and Milton, M. J. T.: High-accuracy gas flow dilutor using mass flow controllers with binary weighted flows, Meas. Sci. Technol., 13, 1138–1145, https://doi.org/10.1088/0957-0233/13/7/323, 2002.
Guillevic, M., Vollmer, M. K., Wyss, S. A., Leuenberger, D., Ackermann, A., Pascale, C., Niederhauser, B., and Reimann, S.: Dynamic–gravimetric preparation of metrologically traceable primary calibration standards for halogenated greenhouse gases, Atmos. Meas. Tech., 11, 3351–3372, https://doi.org/10.5194/amt-11-3351-2018, 2018.
Hall, B. D., Engel, A., Mühle, J., Elkins, J. W., Artuso, F., Atlas, E., Aydin, M., Blake, D., Brunke, E.-G., Chiavarini, S., Fraser, P. J., Happell, J., Krummel, P. B., Levin, I., Loewenstein, M., Maione, M., Montzka, S. A., O'Doherty, S., Reimann, S., Rhoderick, G., Saltzman, E. S., Scheel, H. E., Steele, L. P., Vollmer, M. K., Weiss, R. F., Worthy, D., and Yokouchi, Y.: Results from the International Halocarbons in Air Comparison Experiment (IHALACE), Atmos. Meas. Tech., 7, 469–490, https://doi.org/10.5194/amt-7-469-2014, 2014.
Hall, B. D., Crotwell, A. M., Miller, B. R., Schibig, M., and Elkins, J. W.: Gravimetrically prepared carbon dioxide standards in support of atmospheric research, Atmos. Meas. Tech., 12, 517–524, https://doi.org/10.5194/amt-12-517-2019, 2019.
ISO 21748: Guidance for the use of repeatability, reproducibility and trueness estimates in measurement uncertainty evaluation, available at: https://www.iso.org/standard/71615.html (last access: May 2023), 2017.
ISO 6142-1: Gas analysis – Preparation of calibration gas mixtures–Part 1: Gravimetric method for Class I mixtures, available at: https://www.iso.org/standard/59631.html (last access: January 2023), 2015.
ISO 6145-7: Gas analysis – Preparation of calibration gas mixtures using dynamic methods–Part 7: Thermal mass-flow, available at: https://www.iso.org/standard/ 45471.html (last access: January 2023), 2018.
Kerwin, R. A., Crill, P. M., Talbot, R. W., Hines, M. E., Shorter, J. H., Kolb, C. E., and Harriss, R. C.: Determination of atmospheric methyl bromide by cryotrapping-gas chromatography and application to soil kinetic studies using a dynamic dilution system, Anal. Chem., 68, 899–903, https://doi.org/10.1021/ac950811z, 1996.
Kim, M. E., Kim, Y. D., Kang, J. H., Heo, G. S., Lee, D. S., and Lee, S.: Development of traceable precision dynamic dilution method to generate dimethyl sulphide gas mixtures at sub-nanomole per mole levels for ambient measurement, Talanta, 150, 516–524, https://doi.org/10.1016/j.talanta.2015.12.063, 2016.
Kjellstrom, E.: A three-dimensional global model study of carbonyl sulfide troposphere and the lower stratosphere, J. Atmos. Chem., 29, 151–177, https://doi.org/10.1023/A:1005976511096, 1998.
Kooijmans, L.M.J., Sun, W., Aalto, J., Erkkilä, K., Maseyk, K., Seibt, U., Vesala, T., Mammarella, I., and Chen, H.: Influences of light and humidity on carbonyl sulfide-based estimates of photosynthesis, P. Natl. Acad. Sci. USA, 116, 2470–2475, https://doi.org/10.1073/pnas.1807600116, 2019.
Landau, L. and Lifshitz, E.: Fluid mechanics 2nd edition, Course of theoretical Physics, Volume 6, 51–55, https://doi.org/10.1016/C2013-0-03799-1, 1987.
Langenfelds, R. L., van der Schoot, M. V., Francey, R. J., Steele, L. P., Schmidt, M., and Mukai, H.: Modification of air standard composition by diffusive and surface processes, J. Geophys. Res., 110, D13307, https://doi.org/10.1029/2004JD005482, 2005.
Macé, T., Iturrate-Garcia, M., Pascale, C., Niederhauser, B., Vaslin-Reimann, S., and Sutour, C.: Air pollution monitoring: development of ammonia (NH3) dynamic reference gas mixtures at nanomoles per mole levels to improve the lack of traceability of measurements, Atmos. Meas. Tech., 15, 2703–2718, https://doi.org/10.5194/amt-15-2703-2022, 2022.
Maseyk, K., Berry, J. A., Billesbach, D., Campbell, J. E., Torn, M. S., Zahniser, M., and Seibt, U.: Sources and sinks of carbonyl sulfide in an agricultural field in the Southern Great Plains, P. Natl. Acad. Sci. USA, 111, 9064–9069, https://doi.org/10.1073/pnas.1319132111, 2014.
Mohamad, G. H. P., Coles, G. S. V., and Watson, J.: An automatic low-level gas blender, Trans. Inst. Meas. Cont., 18, 62–68, https://doi.org/10.1177/014233129601800201, 1996.
Montzka, S., Aydin, M., Battle, M., Butler, J., Saltzman, E., Hall, B., Clarke, A., Mondeel, D., and Elkins, J.: A 350-year atmospheric history for carbonyl sulfide inferred from Antarctic firn air and air trapped in ice, J. Geophys. Res., 109, D22302, https://doi.org/10.1029/2004JD004686, 2004.
Montzka, S. A., Calvert, P., Hall, B. D., Elkins, J. W., Conway, T. J., Tans, P. P., and Sweeney, C.: On the global distribution, seasonality, and budget of atmospheric carbonyl sulfide (COS) and some similarities to CO2, J. Geophys. Res.-Atmos., 112, D09302, https://doi.org/10.1029/2006JD007665, 2007.
Nakao, S. and Takamoto, M.: Development of the calibration facility for small mass flow rates of gases and the sonic venturi nozzle transfer standard, JSME Int. J. Ser. B., 42, 667–673, https://doi.org/10.1299/jsmeb.42.667, 1999.
Nara, H., Tanimoto, H., Nojiri, Y., Mukai, H., Machida, T., and Tohjima, Y.: Onboard measurement system of atmospheric carbon monoxide in the Pacific by voluntary observing ships, Atmos. Meas. Tech., 4, 2495–2507, https://doi.org/10.5194/amt-4-2495-2011, 2011.
Nara, H., Tanimoto, H., Tohjima, Y., Mukai, H., Nojiri, Y., Katsumata, K., and Rella, C. W.: Effect of air composition (N2, O2, Ar, and H2O) on CO2 and CH4 measurement by wavelength-scanned cavity ring-down spectroscopy: calibration and measurement strategy, Atmos. Meas. Tech., 5, 2689–2701, https://doi.org/10.5194/amt-5-2689-2012, 2012.
Novelli, P. C., Collins Jr., J. E., Myers, R. C., Sachse, G. W., and Scheel, H. E.: Reevaluation of the NOAA/CMDL carbon monoxide reference scale and comparison with CO reference gases at NASA-Langley and Fraunhofer Institute, J. Geophys. Res., 99, 12833–12839, https://doi.org/10.1029/94JD00314, 1994.
Novelli, P. C., Masarie, K. A., Lang, P. M., Hall, B. D., Myers, R. C., and Elkins, J. W.: Reanalysis of tropospheric CO trends: effects of the 1997–1998 wildfires, J. Geophys. Res., 108, 4464, https://doi.org/10.1029/2002JD003031, 2003.
Protoschill-Krebs, G., Wilhelm, C., and Kesselmeier, J.: Consumption of carbonyl sulphide (COS) by higher plant carbonic anhydrase (CA), Atmos. Environ., 30, 3151–3156, https://doi.org/10.1016/1352-2310(96)00026-X, 1996.
Saito, T., Yokouchi, Y., Stohl, A., Taguchi, S., and Mukai, H: Large emissions of perfluorocarbons in East Asia deduced from continuous atmospheric measurements, Environ. Sci. Technol., 44, 4089–4095, https://doi.org/10.1021/es1001488, 2010.
Sandoval-Soto, L., Stanimirov, M., von Hobe, M., Schmitt, V., Valdes, J., Wild, A., and Kesselmeier, J.: Global uptake of carbonyl sulfide (COS) by terrestrial vegetation: Estimates corrected by deposition velocities normalized to the uptake of carbon dioxide (CO2), Biogeosciences, 2, 125–132, https://doi.org/10.5194/bg-2-125-2005, 2005.
Schibig, M. F., Kitzis, D., and Tans, P. P.: Experiments with CO2-in-air reference gases in high-pressure aluminum cylinders, Atmos. Meas. Tech., 11, 5565–5586, https://doi.org/10.5194/amt-11-5565-2018, 2018.
Seibt, U., Kesselmeier, J., Sandoval-Soto, L., Kuhn, U., and Berry, J. A.: A kinetic analysis of leaf uptake of COS and its relation to transpiration, photosynthesis and carbon isotope fractionation, Biogeosciences, 7, 333–341, https://doi.org/10.5194/bg-7-333-2010, 2010.
Stimler, K., Berry, J. A., and Yakir, D.: Effects of carbonyl sulfide and carbonic anhydrase on stomatal conductance1,[OA], Plant Physiol., 158, 524–530, https://doi.org/10.1104/pp.111.185926, 2012.
Stimler, K., Montzka, S. A., Berry, J. A., Rudich, Y., and Yakir, D.: Relationships between carbonyl sulfide (COS) and CO2 during leaf gas exchange, New Phytol., 186, 869–878, https://doi.org/10.1111/j.1469-8137.2010.03218.x, 2010.
Tanimoto, H., Sawa, Y., Matsueda, H., Yonemura, S., Wada, A., Mukai, H., Wang, T., Poon, S., Wong, A., Lee, G., Jung, J. Y., Kim, K. R., Lee, M., Lin, N. H., Wang, J. L., Ou-Yang, C. F., and Wu, C. F.: Evaluation of standards and methods for continuous measurements of carbon monoxide at ground-based sites in Asia, Pap. Meteorol. Geophys., 58, 85–93, https://doi.org/10.2467/mripapers.58.85 , 2007.
Tera Term project team: Tera Term 4.97 (Version 4.97), 30 November 2017, https://ttssh2.osdn.jp/index.html.en (last access: January 2023), 2017.
Wehr, R., Munger, J. W., McManus, J. B., Nelson, D. D., Zahniser, M. S., Davidson, E. A., Wofsy, S. C., and Saleska, S. R.: Seasonality of temperate forest photosynthesis and daytime respiration, Nature, 534, 680–683, https://doi.org/10.1038/nature17966, 2016.
WMO: Scientific assessment of ozone depletion, 2018, GAW Report 58, World Meteorological Organization (WMO), Global Ozone Research and Monitoring Project, Geneva, Switzerland, https://public-old.wmo.int/en/resources/library/scientific-assessment-of-ozone-depletion-2018 (last access: January 2023), 2018.
WMO: Report of the 20th WMO/IAEA Meeting on carbon dioxide, other greenhouse gases and related measurement techniques, 2–5 September 2019, GAW Report No. 255, https://library.wmo.int/index.php?lvl=notice_display&id=21758 (last access: January 2023), 2020.
WMO: Scientific assessment of ozone depletion, 2022, GAW Report 278, World Meteorological Organization (WMO), Global Ozone Research and Monitoring Project, Geneva, Switzerland, https://www.csl.noaa.gov/assessments/ozone/2022/ (last access: October 2023), 2022.
Wright, R. S. and Murdoch, R. W.: Laboratory evaluation of gas dilution systems for analyser calibration and calibration gas analysis, Air Waste, 44, 428–430, https://doi.org/10.1080/1073161X.1994.10467265, 1994.
Yang, F., Qubaja, R., Tatarinov, F., Rotenberg, E., and Yakir, D.: Assessing canopy performance using carbonyl sulfide measurements, Glob. Chang. Biol., 24, 3486–3498, https://doi.org/10.1111/gcb.14145, 2018.
Yokohata, A., Makide, Y., and Tominaga, T.: A new calibration method for the measurement of CCl4 concentration at 10−10 v/v level and the behavior of CCl4 in the atmosphere, B. Chem. Soc. Jpn., 58, 1308–1314, https://doi.org/10.1246/bcsj.58.1308, 1985.
- Abstract
- Introduction
- Materials and methods
- Long-term stability of COS in high-pressure aluminium cylinders
- Evaluation of dilution performance
- Discussion
- Summary and conclusions
- Data availability
- Author contributions
- Competing interests
- Disclaimer
- Acknowledgements
- Financial support
- Review statement
- References
- Supplement
- Abstract
- Introduction
- Materials and methods
- Long-term stability of COS in high-pressure aluminium cylinders
- Evaluation of dilution performance
- Discussion
- Summary and conclusions
- Data availability
- Author contributions
- Competing interests
- Disclaimer
- Acknowledgements
- Financial support
- Review statement
- References
- Supplement