the Creative Commons Attribution 4.0 License.
the Creative Commons Attribution 4.0 License.
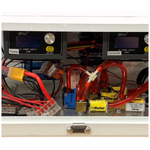
A modular approach to volatile organic compound samplers for tethered balloon and drone platforms
Meghan Guagenti
Darielle Dexheimer
Alexandra Ulinksi
Paul Walter
James H. Flynn III
Situated at a land–sea interface, Houston, Texas, is a national hub for the petrochemical industry and has the second-fastest-growing metropolitan population in the United States. Addressing air quality in this region is uniquely challenging, due in part to its wide range of meteorological conditions (e.g., convection systems and temperature inversions) and continuum of volatile organic compound (VOC) and aerosol sources (e.g., anthropogenic and biogenic). As a result, Houston was chosen as the location for the Department of Energy's Atmospheric Radiation Measurement (ARM)-program-led Tracking Aerosol Convection ExpeRiment (TRACER), which investigated cloud and aerosol interactions in the deep convection over the area. Deployed as a key asset, ARM's tethered balloon system (TBS) was used to investigate questions related to the vertical distributions of aerosols and their formation, including their precursor species volatile organic compounds. Platforms like TBSs and uncrewed aerial vehicles (UAVs) can bridge the vertical gap between ground-based and crewed airplane measurement platforms to focus on near-surface characterization. However, there has been limited effort to modularize and integrate VOC samplers into instrument payloads on both aerial systems. In this study, lightweight and robust VOC samplers were designed and deployed on the TBS and a UAV to collect VOCs in flight. The modular design allowed for scalable adjustments to meet the unique platform requirements and enabled multiple flights per sampling day. Each sampler can autonomously collect VOCs on up to four sorbent tubes for subsequent thermal desorption–gas chromatography–mass spectrometry analysis. The low sampler mass (2.2 kg and 800 g, TBS and UAV, respectively) enables the combination of these VOC samplers with trace gas, aerosol, and meteorological sensors on aerial platforms. These profiles allow us to assess temporal changes in VOC magnitude and composition at multiple locations. Observations from TBS and UAV flights during TRACER are presented and future considerations for sampler design and deployments are discussed.
- Article
(2078 KB) - Full-text XML
-
Supplement
(1391 KB) - BibTeX
- EndNote
Volatile organic compounds (VOCs) play a crucial role in tropospheric chemistry and are emitted from diverse sources, including biogenic and anthropogenic activities. In a city like Houston, Texas, distinguishing between VOC sources can be difficult when traditionally biogenic compounds are used as part of anthropogenic manufacturing processes on an industrial scale. For example, while isoprene is typically classified as a biogenic VOC, emissions in areas like the Houston Ship Channel can reach up to 19 t yr−1 due to activities such as chemical usage, storage, and transportation (as reported by the Texas Commission on Environmental Quality (TCEQ) and stated in Title 30 of the Texas Administration Code, Section 101.10, for Harris County for the year 2022 (data current as of 31 May 2024)). Consequently, isoprene may be regarded as an emission from volatile chemical products in this region. Variations in emission height (i.e., from stacks or as fugitive emissions) may also impact the vertical distribution of species and subsequent atmospheric processing. Understanding the nuances of this processing is a priority for federal and state agencies as VOCs can influence urban atmospheric chemistry by undergoing photooxidation to form secondary organic aerosol (SOA) and ozone (Coggon et al., 2021; Sasidharan et al., 2023).
Atmospheric processing of VOCs can occur rapidly and over relatively short distances, with many compounds exhibiting high reaction rate coefficients with the daytime and nighttime oxidants, resulting in fast atmospheric lifetimes (Atkinson and Arey, 2003; Atkinson, 2000). The urban sources of these VOCs can also vary with time, ranging from known diurnal trends, such as plant-based emissions of isoprene (Park et al., 2011; Bryant et al., 2023) and periods of rush-hour traffic (Shrestha et al., 2022), to pulse event emissions from either biomass burning or industrial activities (Gilman et al., 2015; Shrestha et al., 2023a, b). Moreover, the temporal and spatial (i.e., horizontal and vertical) variability of VOC emissions from both biogenic and anthropogenic sources can influence the urban VOC composition through changes in various factors, including source-related aspects (i.e., type, strength, and proximity to), meteorological conditions (i.e., time of day or season), and plume mixing (i.e., transport and mixing of emissions from upwind sources, such as biomass burning). As urban emission sources evolve, the spatial and temporal distributions of VOCs can be altered and affect their atmospheric processing, thereby influencing the formation of secondary products (e.g., ozone and SOA).
Traditionally, VOC observations have been made from fixed, ground-based locations and have been instrumental in the long-term monitoring and characterization of surface-level species. Uncrewed aircraft have been employed for spatial and vertical assessments in lower regions of the troposphere, such as in the atmospheric boundary layer (Müller et al., 2014; Permar et al., 2021; Dreessen et al., 2023). Sampling payloads on aircraft can include a wide range of instrumentation, including several different types of monitors for aerosols, trace gases, and VOCs. For example, payloads may include surface-facing lidars, aerosol concentration monitors, and VOC concentration and composition instrumentation that complement ground-based suites to help characterize the vertical column near the earth's surface (0–1500 m above ground level (a.g.l.)). This section of the atmosphere that contains the biosphere is a dynamic area subject to abrupt changes in composition due to ground-based emissions as well as meteorological influences (i.e., convection). However, near-surface measurements, including VOCs, remain largely under-characterized due to several limitations such as financial and logistical challenges and a lack of commercially available VOC samplers for aerial platforms.
Aerial platforms, such as tethered balloon systems (TBSs) and uncrewed aerial vehicles (UAVs; i.e., drones), have been utilized recently to bridge the gap between surface and crewed aircraft atmospheric measurements at low altitudes. The platforms differ in cost, operational logistics, payload capacity, maximum flight ceiling, and in-flight maneuverability. A review of vertical sampling technologies for VOCs highlighted the limitations of available chemically sensitive sensors for aerial platforms and noted the UAV's potential to carry payloads consisting of multiple sensors and provide high spatiotemporally resolved data (Dieu Hien et al., 2019). TBS-based VOC observations date back to Amazonian observations in the 1980s, which were built on and deployed in more urbanized settings periodically throughout the 1990s–2000s. In these studies, researchers used multiple VOC sampling units per flight (Andronache et al., 1994; Davis et al., 1994; Helmig et al., 1998; Greenberg, 1999; Spirig et al., 2004; Greenberg et al., 2004). However, each unit contained a single sample collection vessel, such as a sorbent tube, Teflon bag, or canister. In order to collect multiple samples per flight, several units were distributed along the tether, each controlled by individual timers to construct vertical profiles. Currently, the commercially available options for VOC sampling have been primarily designed for ground-based sampling, including personal monitoring and long-term monitoring applications. These systems were not designed for flight-based measurements or to be integrated into flight platforms with additional sensors by meeting the weight, size, and power limits. Alternatively, whole air samplers have recently been deployed on UAV platforms, enabling up to 15 samples to be collected per flight (Asher et al., 2021; Leitner et al., 2023). Recent advancements in sorbent tube VOC samplers have focused on providing low cost, portable samplers (Hurley et al., 2023) and UAV-based VOC samplers with multiple tube sampling capabilities (Chen et al., 2018; Batista et al., 2019; McKinney et al., 2019; Asher et al., 2021; Li et al., 2021; Leitner et al., 2023; Yang et al., 2023; Zhai et al., 2025). Many of these advancements have been made possible through miniaturization of key components (e.g., size and power requirements), such as computers, pumps, values, and relays. However, a modular sampling system capable of functioning independently to collect multiple VOC samples per flight on a TBS has not been designed and deployed.
The goals of this work were to (1) design and fabricate a modular sampler capable of programmable VOC collection on tethered balloon flights; (2) integrate the developed sampler into the ARM TBS payload via deployment with meteorological, aerosol, and ozone instrumentation; and (3) customize the modular sampler to enable integration into a UAV power system for VOC collection at the Houston Ship Channel. The VOC sampler design was optimized to maximize flight time with other sensors (i.e., trace gas and aerosol monitors) and participate in multiple flights per day. The standalone unit presented here can be programmed to capture up to four VOC samples per flight and be easily expanded using the approach described below, allowing for various vertical profiling sampling approaches (i.e., continuous sampling during ascent/descent or measurements at discreet altitudes). Observations presented herein were collected during the Tracking Aerosol Convection ExpeRiment (TRACER) in Houston, Texas, in the summer of 2022. Sampler design and optimization, including computer programming to control sampling, system ruggedization, and parts and components, are described in the following sections.
2.1 Tethered balloon platform
The TBS platform utilized here is owned by the U.S. Department of Energy and was operated by a team of trained professionals out of Sandia National Laboratories in support of TRACER goals (TRACER-Tethersonde, https://www.arm.gov/research/campaigns/amf2021tracer-ozone, last access: 12 May 2024). The operation and logistics have been described in detail elsewhere (Dexheimer et al., 2024). In brief, TBS flights can reach a maximum altitude of 1.5 km a.g.l. when the weather permits and carry a maximum payload of 33 kg via a tether controlled by a winch system. In support of the project goals, the TBS payload typically consisted of commercial, real-time aerosol and meteorological sensors and select trace gas instrumentation. VOC data from select flights are presented here and can be found through the ARM Data Discovery portal, along with all ARM data used in this study (https://adc.arm.gov/discovery/#/, last access: 12 May 2024). The commercial sensors integrated into the ARM TBS platform included a modified iMet-4 RSB radiosonde to collect temperature, pressure, relative humidity, and GPS altitudes using an external power source (International Met Systems, Inc., MI, USA). In addition, particle size distributions were measured using a portable optical particle spectrometer (POPS; Handix Scientific LLC, CO, USA; 135–3615 nm), and ozone concentrations were provided via an electrochemical concentration cell (ECC) ozonesonde (Model 2Z, En-Sci, CO, USA). VOCs were collected on the descent leg of the flights, and the TBS loitered at the desired altitude for the 20 min sampling period.
2.2 UAV platform
The hexacopter UAV (Aurelia X6 Pro, UAV Systems International, NV, USA) used in this study was owned by the University of Houston (UH) and operated by a licensed professional from UH in support of the TRACER sub-campaign TRACER-Air Quality 2 (TRACER-AQ2). The UAV had a payload capacity of 6 kg and was flown at a maximum altitude of 120 m a.g.l. An ECC ozonesonde and iMet-1 RSB were flown in addition to the VOC sampler on select flights (Fig. S2 in the Supplement). To minimize sampler mass, the frame was constructed using miniature T-Slotted framing and fittings, with walls made from high-flow aluminum perforated sheets. See the Supplement for the housing component's part numbers. VOCs were collected at the maximum altitude (120 m a.g.l.) and at 60 m a.g.l. to explore vertical gradients. Previous studies have investigated the air velocity distribution around UAVs and found the air flushing time below the UAV is much faster (i.e., seconds) than the VOC sampling timescale (i.e., 10 min); therefore the VOC samples may represent an air parcel extending up to ±5 m surrounding the sampling height (Ventura Diaz and Yoon, 2018; McKinney et al., 2019). As such, a ±5 m was applied to the sampling altitudes presented in the text associated with VOC measurements.
2.3 Sampling campaigns
The platforms were operated in support of overall TRACER goals as part of two different sub-campaigns, TRACER-Tethersonde (https://www.arm.gov/research/campaigns/amf2021tracer-ozone, last access: 7 January 2024) and TRACER-AQ2. The TBS was deployed at the ancillary (i.e., rural) site (29.328, −95.741) ∼50 km southwest of downtown Houston, and the UAV was operated at the San Jacinto Battleground State Historic Site (29°44′56′′ N, 95°4′49′′ W; a site map is shown in Fig. S1) near the Houston Shipping Channel (i.e., urban-influenced). TBS flights were conducted monthly for 2-week periods from June to September 2022 and the UAV was flown periodically during August and September 2022.
2.4 VOC sampler
The modularity of the VOC sampler described here enabled deployments on two different aerial platforms. The internal components described below were used on each platform, and the VOC sampler flown on the TBS and a schematic of the sample flow path, data communication path, and power connections are shown in Fig. 1. The TBS sampler housing is a carbon fiber material that was 3D-printed (58.4 × 16.5 × 12.7 cm (L × W × H)) with three user-access ports, including a main opening along the length of the sampler and two 15.2×15.2 cm openings on the top for access to the power source and the sorbent tubes (Fig. S3). The housing also has two fans built into the system to cool the computer and other electronics while in flight. This adjustment allows for extended operational deployments, even in environments with elevated ambient temperatures. Sampling tubes were secured during flights using grommets on the unit housing, which also allowed easy access during tube exchanges. The system was powered using a 21 V DC battery and three voltage regulators to supply sufficient voltage to the internal components (i.e., pump, valves, and computer). The computer (UDOO Bolt, UDOO, Siena, Italy) controlled the Arduino-operated microcontroller and was programmable to automate sample collection of up to four tubes in flight. The automation code was written in the open-source software Arduino IDE, and a copy of the code is provided in the Supplement. During each flight, an indicator in the Arduino software signalled the completion of the sampling plan to provide an in-field indication of successful sample collection. The sampler mass of 2.2 kg and 800 g, for the TBS and UAV versions, respectively, was optimized for use on each platform. A table with the internal components and software used in this study is provided in the Supplement (Table S1). Many of the components within this system are readily available off-the-shelf, and their modular design allows for convenient upgrades to various versions, catering to the specific requirements of users. Before each sampling day, a flow calibration was conducted on each sorbent tube to account for potential variability among the tubes and to ensure the flow remained at 0.1±0.002 L min−1, as measured using a flowmeter (Model 5200, TSI Inc, MN, USA). When adjustments to the sample flow rate were necessary, users modified the pump voltage (Model E242-12, Parker, OH, USA) using the digital voltage regulator.
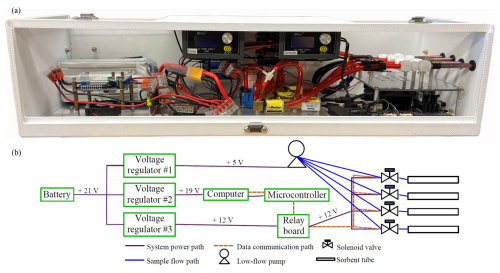
Figure 1A side view of the TBS VOC sampler with the top and side access panels open (a) and a schematic of the modular sampler, including sample flow, electrical, and data communication paths (b). Additional photos of the finalized designs for both the TBS and UAV samplers are in the Supplement (Figs. S1 and S2).
2.5 VOC sample collection and chemical analysis
2.5.1 VOC collection on sorbent tubes
Air samples were collected using dual-bed sorbent tubes packed with Tenax TA and Carbograph 5TD (Markes International, Bridgend, UK) to capture C–C32 organic compounds. After conditioning at 280 °C for 60 min, tubes were capped with brass compression fittings to isolate sorbents from ambient air and placed in clean 50 mL conical centrifuge tubes as a secondary layer of separation. Prior to sampling, sorbent tubes were kept in cold storage at 5 °C for up to 2 weeks and transferred to double-walled stainless-steel coolers to maintain sample integrity during transport to the sampling location. In the field, the tubes were installed into the sampler and attached to the sampling lines via Teflon compression fittings. During each sampling flight, samples were either collected automatically based on a pre-written code (i.e., TBS operations) or controlled manually by the pilot (i.e., UAV operations) to draw ambient air through a sorbent tube at a flow rate of 0.1 L min−1 for 10 min on UAV flights and 20 min on TBS flights (total sample volumes of 1 and 2 L, respectively). The configuration of valves within the samplers allowed the operators to sample each tube independently or collect paired samples. After the flights, tubes were recapped and placed in short-term storage before transport back to the lab in double-walled stainless-steel coolers. The samples were placed in long-term storage at 5 °C for up to 2 weeks before chemical analysis at the Baylor University Mass Spectrometry Center.
2.5.2 Thermal desorption–gas chromatography–tandem mass spectrometry analysis
VOCs were analyzed using a thermal desorption–gas chromatography–tandem mass spectrometry system (TD-GC-MS/MS, TD-100xr, Markes International, Bridgend, UK, paired with a Thermo Scientific TRACE 1310 coupled to a TSQ 8000 Evo, MA, USA). A similar methodology has been employed in previous studies (Ribes et al., 2007; Gallego et al., 2012; Schieweck et al., 2018; McKinney et al., 2019; Helin et al., 2020). Here, volatile species were desorbed from the tubes at 250 °C for 1 min under a constant flow of helium at 1 mL min−1. The desorbed samples were transferred along a 150 °C flow path and were either split to the cold trap for analyte preconcentration at 20 °C prior to GC injection or recollected back onto the sorbent tube for reanalysis. The cold trap was heated at a rate of 40 °C s−1, and the desorbed sample was injected via splitless mode into the GC for chemical separation using a DB-624 column (60 m ×0.32 mm ID ×1.8 µm, J&W Scientific, CA, USA) as the GC was heated from 40 to 255 °C (total GC-MS/MS runtime: 36 min).
Selective reaction monitoring (SRM) was used to quantify all target analytes after being ionized via electron impact at 70 eV with an ion source temperature of 250 °C. Argon was used in the collision cell, and the retention times and ratios used for ion transitions and quantification of each species can be found in Table S3 in the Supplement. The samples were also reanalyzed using a full scan (35 to 550 ) mode for untargeted analysis.
To quantify the target analyte concentrations, a seven-point calibration curve was produced across a range of 10 ppb to 12 ppm. A minimum of five points were used to describe each analyte's dynamic linear range and all analytes exhibited R2 values greater than 0.99. Neat chemical standards from Sigma Aldrich were placed in dichloromethane at concentrations ranging from 150 to 1000 ng µL−1 (Table S2, Sigma Aldrich, MA, USA). The standard solutions were combined and diluted to make seven calibration solutions. The calibration solutions were spiked onto sorbent tubes under a flow of 0.5 mL min−1 of N2 for 5 min (Chen et al., 2018). A digital syringe with a precision of 0.5 % was utilized to increase reproducibility when spiking 1 µL of calibration solutions (DS80135, Hamilton Company, NV, USA). Quality assurance and quality control were monitored during sample analysis by running a continuing calibration verification (CCV) standard solution at regular intervals (approximately every fourth injection). CCV standard solutions included all analytes at the middle point of the calibration curve. Analyte recoveries beyond ±40 % from CCV analyses required a new calibration curve to be run. All calibration tubes contained the same sorbents and were run using the same chemical analysis methods as field samples. Following chemical analysis, the samples underwent a blank correction to account for artifacts from passive sampling, travel, storage prior to analysis, and potential laboratory contamination. A 30 % error was applied to the VOC measurements to reflect the variability in the sampling (e.g., flow rate accuracy) and analysis methods (e.g., sampling efficiency, sorbent tube desorption, and instrumental response variability). Specifically, the flow meter used to calibrate the sample flow has an error of 2 %.
3.1 Performance test flights
Following in-lab design and construction, a prototype of the VOC sampler underwent TBS test flights in the spring of 2022 at the ARM Southern Great Plains user facility (https://www.arm.gov/capabilities/observatories/sgp, last access: 15 April 2025). Flight plans were focused on evaluating the performance and functionality of the sorbent tube collection system, including (1) design features such as sampler housing and internal hardware, (2) mid-flight sample automation capabilities, and (3) accessibility of the system for routine maintenance and troubleshooting. Prior to test flight launches, the user adjusted the pre-written code to reflect the flight's intended sample collection. The code initiation was synchronized to match the sampling strategies of the VOC sorbent tube collection with the TBS platform's flight plan. To conserve power and only sample during predetermined times, the computer did not provide power to the pumps until the TBS was expected to reach the first sampling altitude. Once at altitude, the pump and associated valves were powered for sample collection on a specific tube and then turned off while in transit to the next sampling altitude. During sampling, each sorbent tube was used once, while the process (i.e., powering the pump and opening and closing a unique combination of values) was repeated sequentially until all samples were collected. Note that these sequential samples provide VOC measurements along a vertical TBS or UAV flight, where VOC measurements are offset temporally during the descent leg of the flight. After the final collection period, the pump remained unpowered, and an output message was displayed to indicate the successful execution of the flight algorithm.
Initial flights of the prototype revealed both successful aspects and limitations of the tested features. While the sampler housing and electrical connections remained intact through multiple flights, the original hardware loosened and allowed the internal components to jostle inside the housing and compromised sampling capabilities. After replacing the hardware with socket head bolts, functionality tests of in-flight automation using a microcontroller and Arduino software were successful. During maintenance to replace the hardware, concerns were identified with the sampler's ease of use. Specifically, it was difficult to access key internal components due to the lack of access ports. This limitation could also affect the operator's ability to routinely exchange tubes or troubleshoot electrical or hardware issues that arise in-field. Therefore, two panels were added along the length of the final design to facilitate top-down access for users, particularly above the sorbent tube and the power source areas. Houston-based test flights also highlighted heat-related electrical failures in the extreme ambient conditions (e.g., >38 °C) of the TRACER operations. To prevent the computer and electronics from overheating and powering down mid-flight, additional ventilation modifications were made to the final design's housing.
3.2 TBS and UAV sample collection
The samplers designed herein were successfully used to collect VOCs on the ARM TBS and a UAV during the TRACER intensive operating period (IOP). Across 10 TBS flights at the TRACER ancillary site, 19 VOC samples were collected during the August deployment. Morning and afternoon flights were conducted to capture temporal changes in all measured species and aligned with other TBS TRACER-related experimental designs. For example, seven TBS flights occurred on 4 August 2022, from ∼ 14:00 to ∼ 22:00 UTC, and a combination of VOC, meteorological, aerosol, and ozone monitors were successfully flown on each flight (Fig. 2). Sequential VOC samples were collected on three of the 4 August flights, including a morning flight (flight no. 2, sample 1), a midday flight (flight no. 3, samples 2 and 3), and a late-afternoon flight (flight no. 6, samples 4, 5, and 6). These flights highlight the capability of this system to successfully collect VOCs on TBS flights, particularly the midday and afternoon launches that collected multiple samples per flight. Further, a single VOC sampling unit successfully worked in tandem with the other monitors to generate three vertical profiles throughout the day and captured changes in all measured species, including VOC magnitude and composition (see Sect. 3.3).
At the San Jacinto Battleground State Historic Site, meteorological parameters, ozone, and four VOC samples were collected across two UAV flights on 13 September 2022 (Fig. 3). The UAV sampler design demonstrates the scalability of the modular VOC sampler, and the flights showcase the utility of pairing the internal power and communication features of the UAV with the lightweight, robust components of the sampler to successfully collect VOCs. In this setup, the UAV control software initiated VOC collection by activating relays within the UAV system. These relays supply power to the sampling pump and the valves to control sampling timing and selecting which sorbent tube to sample. Additionally, these flights highlight the feasibility of sub-hourly VOC collection within the first 125 ± 5 m a.g.l. and were enabled via a short (<15 min) exchange time for the tubes and UAV battery replacement. The fast turnaround time between flights provides an opportunity to capture temporal variation in chemical profiles within the dynamic near-surface region that can be quickly influenced by meteorological factors or emission shifts, for example, the temporal evolution of secondary product production (e.g., monitoring photochemical ozone production at sunrise) or assessing temporal variability of VOCs at the community level related to local industrial activities. This increased temporal resolution of measurements would be particularly important to bolster estimates of local emissions and potential exposures, which are usually calculated using the annual averages reported to state agencies.
One of the key limitations to the commercially available options for sorbent tube VOC sampling in ground and aerial applications is the lack of sampling control. For instance, an automatic sampler from Markes International (MTS-32) has the capacity to sample up to 32 sorbent tubes but has limited flexibility to conduct complex sampling. The large mass of the MTS-32 (>5 kg) makes it unsuitable for use in aerial sampling packages on UAVs or TBSs. As a ground-based system, the MTS-32 can only be programmed with a fixed sample duration (e.g., 10 min) for all 32 tubes, preventing any adjustments to the sampling strategy mid-operation. Additionally, the current version cannot be connected to a computer for remote operation. With the computer and microcontroller utilized in the sampler design discussed here, users can perform more complex sampling strategies. For example, metadata from secondary sensors (e.g., ozone concentrations) may direct the computer to sample from one resin tube containing specific resins over another. Furthermore, the previous generation of aerial VOC samplers lacked manual control of sampler functions from the tablet-based UAV control software. In this study, the real-time communication between the UAV and the software enabled researchers to adapt the sampling plan in response to real-time indicators such as observations made by the ground crew and enables future sampling to potentially respond to sensors on board, as mentioned previously.
Additional considerations were made regarding the sampler housing on both platforms, opting for materials robust enough to accommodate the internal components, adaptable for in-field adjustments, and lightweight to minimize overall sampler weight. Another key consideration was the internal component's functionality across various conditions, including extreme surface temperatures and altitude changes. Therefore, we sourced parts capable of operating within our anticipated temperature and pressure ranges. With the straightforward modification of vents to the housing, the system operated reliably across multiple flights, even under challenging conditions such as high humidity (>90 %) and temperature (range 28–38 °C observed on 4 August, Table S4 and Fig. 2a). In addition, considering the TBS system's power consumption during sampling (2.4 A) and battery capacity, we estimate the system could conduct continuous sampling for 2.2 h before requiring replacement, equating to 13 consecutive VOC samples (10 min collection time). When on board the UAV, the sampling system utilized the internal batteries and consumed 1 Ah of power during VOC collection, representing 4 % of the capacity (two 21 000 mAh, 22.2 VDC batteries).
As part of the TRACER experimental design, two sampling sites were selected to characterize the broad range of environmental and aerosol regimes within the region (e.g., the main site, near UAV flight location, and the ancillary site, TBS flight location). The main site was chosen to characterize the variation in aerosol properties stemming from urban, industrial, and refinery sources in the Houston area near the Houston Ship Channel. The ancillary site was 50 km southwest of the urban core and represented a background area expected to experience more pristine aerosol conditions. TBSs typically operate in rural areas with extensive unobstructed airspace, which allows TBS flights to reach greater heights than urbanized operations. During TRACER, the ancillary site helped to assess changes in aerosol and VOC background concentrations and composition to better characterize the transport and mixing of background and urban emissions. In addition, the UAV measurements conducted along the heavily industrialized Houston Ship Channel (near the main site) provided vertical profiles of volatile species near known emissions sources. The aerial data can help inform manufacturing emission factors specific to this distinct setting, which is important for the surrounding communities. These areas can experience direct impacts from changes in emissions due to shifts in industrial activities, thus driving environmental inequalities. Future projects could integrate the capabilities of the TBS and UAV platforms to enhance scientific investigations. For example, sampling near an emission source with a UAV and at a downwind receptor location with a TBS would enable the assessment of hotspots of atmospheric processing within the vertical profile as the plume is transported across an airshed. This approach would help assess air mass dynamics, especially during transitional periods throughout the day to track vertical mixing related to spatial and temporal changes during transport.
3.3 Vertical VOC mixing ratios
TBS flights on 4 August flew an instrumentation suite of VOCs, ozone, aerosol size, and meteorological sensors. VOCs were identified in all samples, and their composition is shown in Fig. 2b. The total measured VOC mixing ratios were similar on the morning and midday flights (average: 2.4±0.7 and 3.2±1.0 ppbv, respectively), and increased by a factor of 2 on the evening flight (flight no. 6, average: 7.3±2.2 ppbv, Table S5). The select VOC mixing ratios peaked at 10.3±3.1 ppbv on the mid-afternoon flight (flight no. 6, sample no. 4) and decreased in samples no. 5 and 6 as the platform neared the surface. Isoprene was the predominant component of the VOC composition during all flights at the background site. On flight no. 6 (sample no. 4), isoprene peaked at 8.7±2.6 ppbv, following the known daytime trend of increasing mixing ratios coincident with increasing solar radiation. These isoprene mixing ratios are consistent with previous observations of vertical isoprene gradients in the Amazon. For example, aerial mixing ratios ranging from 0.6–11 ppbv have been reported on aircraft and balloon studies, reflecting the variability in the vertical column across different locations (Greenberg et al., 2004; Karl et al., 2007; Jardine et al., 2016; Liu et al., 2018; Batista et al., 2019). Monoterpenes, including α-pinene, β-pinene, and limonene, accounted for 10 % of the daily VOC composition. The biogenic emissions of these reactive compounds from vegetation depend on the type of vegetation, temperature, sunlight intensity, and responses to changes in their natural environment (e.g., storms or droughts) (Kim, 2001; Guenther et al., 2012; Steiner, 2020). In addition to biogenic sources, these compounds can also be emitted from the usage and manufacturing of volatile chemical products (Gkatzelis et al., 2021a, b). These consumer-based anthropogenic sources highlight the complexity of isoprene and monoterpene emissions in urban regions, which can influence their subsequent secondary chemistry to form SOA. These processes can ultimately impact regional land–atmosphere interactions, particularly in areas with a combination of natural, managed, and urban landscapes, such as the southeastern United States. Anthropogenic VOCs (e.g., benzene, toluene, ethylbenzene, and the xylene isomers (BTEX)) were identified as well on TBS flights, primarily below 0.3 km a.g.l., consistent with ground-based automotive sources. The observed BTEX mixing ratios are similar to daytime observations from 2021 TRACER-AQ measurements at the San Jacinto Battleground site, situated approximately 1 km from the UAV operations in this study (Shrestha et al., 2023a). In contrast to the exceptionally high nighttime measurements in 2021, BTEX remained below 1 ppbv during our observations. This suggests the absence of industrial influence at the site location during this sampling period. Throughout the day, ozone concentrations increased gradually yet remained consistent across different sampling altitudes. The low (≤30 ppbv) ozone concentrations were consistent with a background location upwind from primary emission sources. Aerosol observations prior to flight no. 6 showed minimal variability, with concentrations below 250 cm−3 on all flights and primarily consisting of 0.13–0.33 µm aerosols (>97 % of aerosol size composition). However, the peak aerosol concentration of 1900 cm−3 aligns temporally with the largest VOC mixing ratio, which indicates that a change in both the gas and particle phase was captured with this payload. It is important to emphasize that the analytes quantified in this study represent only a select subset of the total VOCs present in the atmosphere.
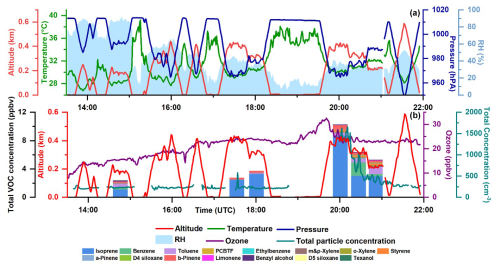
Figure 2Time series of measured parameters from TBS flights on 4 August 2022. (a) TBS flight altitude, ambient temperature, pressure, and relative humidity (RH) observations. (b) VOC flight times (20 min samples, scaled to size) and chemical composition, TBS altitude, ozone measurements, and aerosol measurements on all three flights.
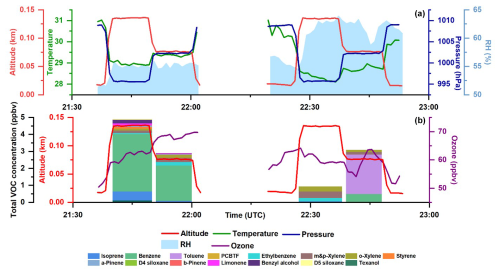
Figure 3Time series of measured parameters from UAV flights on 13 September 2022. (a) UAV flight altitude, ambient temperature, pressure, and relative humidity (RH) observations. (b) VOC flight times (10 min samples, scaled to size) and chemical composition, UAV altitude, and ozone measurements on two flights.
UAV measurements near the Houston Ship Channel also captured the temporal variability of VOC mixing ratios and composition (Fig. 3). Peak select VOC mixing ratios were observed on the first flight (5.0 ppbv) at 120±5 m a.g.l., followed by a decrease to 2.9±0.9 ppbv observed at 60±5 m a.g.l. (Table S6). On the second flight, the select VOC mixing ratios were 0.9±0.3 ppbv at 120±5 m a.g.l. and 3.1±0.9 ppbv at 60±5 m a.g.l. Benzene, ethylbenzene, and the xylene isomers primarily contributed to the VOC composition (>70 %) on all flights. Isoprene, α-pinene, and limonene were also detected on the first flight, which could be related to either the biogenic sources (e.g., oak and pine trees) within the San Jacinto Battleground State Historic Site or local manufacturing facilities that surround the park. Ozone concentrations ranged from 50–70 ppbv within the 1.5 h of flights, almost 2 times the concentrations observed at the rural TBS site. Ambient VOC mixing ratios depend on many conditions, including proximity to and strength of emission sources, photochemical processing, meteorological parameters, time of day, and temperature, which can vary on spatial and temporal scales. These field results demonstrated the application of the VOC sampler on the TBS and UAV; more interpretation and analysis are needed to understand the VOC measurements and processes in subsequent papers, including the incorporation of trace gas, aerosol, and meteorological datasets.
As aerial platforms continue to be deployed to address a wide range of scientific questions, the modular samplers presented here enable the study of VOC emissions for a variety of sampling strategies. The modular structure of the presented sampler allows the user to adjust the size and capacity for their experimental needs. For example, the sorbent tube quantity could be scaled up to increase the number of samples per flight, with minimal increases to the power draw and sampler mass. A sorbent tube, valve, tubing, and a Teflon compression fitting have a mass of approximately 75 g, representing 3 % and 9 % of the TBS and UAV sampler mass, respectively. This adjustment would allow users to enhance vertical profiles by collecting samples on the ascent and descent legs or explore temporal variability at a select altitude by loitering and routinely collecting samples. These observations can help fill knowledge gaps in VOC chemistry and secondary product formation aloft, particularly in the dynamic near-surface area that current ground-based and crewed aerial platforms struggle to characterize. Furthermore, these chemically speciated, vertically resolved measurements will help improve the chemical modeling associated with the vertical transport and processing of VOCs.
The aerial operations described here were conducted as part of two independently operated TRACER sub-campaigns. In this study, the automated sampler successfully collected six VOC samples on sorbent tubes during ARM TBS flights in extreme ambient conditions (Table S4). Subsequently, the modular sampler was downsized for UAV flights and collected multiple sorbent tube samples across successive flights. The modular design of the system allowed for a straightforward integration into the UAV's power system, which made the sampler easy to install on the platform. The sample automation within this design reduces the number of necessary units per flight and opens the payload capacity to include sensors such as aerosol (e.g., ARM's POPS, STAC, IcePuck), trace gas, and meteorological measurements. The detailed profiles collected on the TBS are crucial for understanding hotspots of atmospheric processing within the profiles, which may arise from surface-based sources or descend from aloft. These profiles help to characterize factors influencing aerosol size, chemical composition, and ice nucleating properties.
The modular sampler introduced herein has a flexible design with several adjustable components that can be specialized for future sampling designs. Some examples are given as follows:
-
The internal battery could be scaled down to more widely available batteries (e.g., name brand 20 V power tool battery) to further reduce sampler mass on flights, or the power source could be changed to a shore power connection for a long-term, ground-based deployment.
-
The computer could be upgraded with a Wi-Fi capability to enable remote communication for a user to control sampling at a long-term ground-based sampling site. The sorbent tube measurements could be a standalone measurement, or they could pair with co-deployed real-time instrumentation to provide enhanced chemical speciation.
-
Additional sensors or monitors (e.g., ozone, pressure, GPS) could be added to the system and provide real-time measurements to the computer via Bluetooth communication. These sensors could be integrated into a data-driven sampling strategy that allows for a more targeted VOC analysis in response to in situ conditions.
-
VOC collection could be customized by altering the sorbents within the tubes, incorporating an ozone or particle scrubber before the tube inlet, or extending VOC sampling to different environmental matrices (e.g., soils).
-
The modular approach could also be downsized further to serve as a single-tube sampler. The standalone unit would enable constant flow at a rate that is determined by the input voltage. The sampling would also require minimal battery capacity for a 10 min sample and be easily transported between sampling sites in a durable, protective case. For a picture of such a sampler utilizing these components, refer to the Supplement (Fig. S3).
The robust and user-friendly design can be adapted to extreme climate conditions, sampling methodologies, and platforms and pair with additional sensors to assess VOC chemistry atmospheric processing aloft.
The automation code was written in the open-source software Arduino IDE, and a copy of the code is provided in the Supplement.
TBS VOC data from select flights are presented here and can be found through the ARM Data Discovery portal, along with all ARM data used in this study (https://doi.org/10.5439/1995524, Walter et al., 2025a; https://doi.org/10.5439/2007751, Walter et al., 2025b). The UAV VOC data are available upon request from the TCEQ.
The supplement related to this article is available online at https://doi.org/10.5194/amt-18-2125-2025-supplement.
MG and SU conceptualized and developed the presented sampler. SU and JHF were responsible for the study's funding and supervision. MG was responsible for development of the chemical analysis methods, sample collection and offline analysis, data processing/interpretation, and the drafting of the manuscript. SU edited the manuscript. PW was responsible for ozonesonde data collection and processing from tethered balloon and UAV flights. DD aided in sampler development and supervised tethered balloon sampling flights. AU piloted the UAV flights and supervised VOC collection. All authors have read and agreed to the published paper.
The contact author has declared that none of the authors has any competing interests.
The contents, findings, opinions, and conclusions are the work of the authors and do not necessarily represent findings, opinions, or conclusions of the TCEQ.
Publisher's note: Copernicus Publications remains neutral with regard to jurisdictional claims made in the text, published maps, institutional affiliations, or any other geographical representation in this paper. While Copernicus Publications makes every effort to include appropriate place names, the final responsibility lies with the authors.
The authors thank the TRACER site operators and TBS operators for their dedication and support. The authors also thank Casey Longbottom for sharing his expertise and 3D-printing the TBS sampler housing. They also wish to acknowledge the resources and instrumentation at the Baylor Mass Spectrometry Center.
The research was supported in part by the U.S. Department of Energy's Atmospheric Science Research, an Office of Science Biological and Environmental Research program, under DOE/SC-ARM-23-007. Funding for this work was also provided by the Texas Commission on Environmental Quality (TCEQ; project no. 582-21-22317-015).
This paper was edited by Eric C. Apel and reviewed by two anonymous referees.
Andronache, C., Chameides, W. L., Rodgers, M. O., Martinez, J., Zimmerman, P., and Greenberg, J.: Vertical distribution of isoprene in the lower boundary layer of the rural and urban southern United States, J. Geophys. Res., 99, 16989, https://doi.org/10.1029/94jd01027, 1994.
Asher, E., Hills, A. J., Hornbrook, R. S., Shertz, S., Gabbard, S., Stephens, B. B., Helmig, D., and Apel, E. C.: Unpiloted Aircraft System Instrument for the Rapid Collection of Whole Air Samples and Measurements for Environmental Monitoring and Air Quality Studies, Environ. Sci. Technol., 55, 5657–5667, https://doi.org/10.1021/acs.est.0c07213, 2021.
Atkinson, R.: Atmospheric chemistry of VOCs and NOx, Atmos. Environ., 34, 2063–2101, https://doi.org/10.1016/S1352-2310(99)00460-4, 2000.
Atkinson, R. and Arey, J.: Atmospheric Degradation of Volatile Organic Compounds, Chem. Rev., 103, 4605–4638, https://doi.org/10.1021/cr0206420, 2003.
Batista, C. E., Ye, J., Ribeiro, I. O., Guimarães, P. C., Medeiros, A. S. S., Barbosa, R. G., Oliveira, R. L., Duvoisin, S., Jardine, K. J., Gu, D., Guenther, A. B., Mckinney, K. A., Martins, L. D., Souza, R. A. F., and Martin, S. T.: Intermediate-scale horizontal isoprene concentrations in the near-canopy forest atmosphere and implications for emission heterogeneity, P. Natl. Acad. Sci. USA, 116, 19318–19323, https://doi.org/10.1073/pnas.1904154116, 2019.
Bryant, D. J., Nelson, B. S., Swift, S. J., Budisulistiorini, S. H., Drysdale, W. S., Vaughan, A. R., Newland, M. J., Hopkins, J. R., Cash, J. M., Langford, B., Nemitz, E., Acton, W. J. F., Hewitt, C. N., Mandal, T., Gurjar, B. R., Shivani, Gadi, R., Lee, J. D., Rickard, A. R., and Hamilton, J. F.: Biogenic and anthropogenic sources of isoprene and monoterpenes and their secondary organic aerosol in Delhi, India, Atmos. Chem. Phys., 23, 61–83, https://doi.org/10.5194/acp-23-61-2023, 2023.
Chen, J., Scircle, A., Black, O., Cizdziel, J. V., Watson, N., Wevill, D., and Zhou, Y.: On the use of multicopters for sampling and analysis of volatile organic compounds in the air by adsorption/thermal desorption GC-MS, Air Quality, Atmosphere & Health, 11, 835–842, https://doi.org/10.1007/s11869-018-0588-y, 2018.
Coggon, M. M., Gkatzelis, G. I., Mcdonald, B. C., Gilman, J. B., Schwantes, R. H., Abuhassan, N., Aikin, K. C., Arend, M. F., Berkoff, T. A., Brown, S. S., Campos, T. L., Dickerson, R. R., Gronoff, G., Hurley, J. F., Isaacman-Vanwertz, G., Koss, A. R., Li, M., Mckeen, S. A., Moshary, F., Peischl, J., Pospisilova, V., Ren, X., Wilson, A., Wu, Y., Trainer, M., and Warneke, C.: Volatile chemical product emissions enhance ozone and modulate urban chemistry, P. Natl. Acad. Sci. USA, 118, e2026653118, https://doi.org/10.1073/pnas.2026653118, 2021.
Davis, K. J., Lenschow, D. H., and Zimmerman, P. R.: Biogenic nonmethane hydrocarbon emissions estimated from tethered balloon observations, J. Geophys. Res., 99, 25587, https://doi.org/10.1029/94jd02009, 1994.
Dexheimer, D., Whitson, G., Cheng, Z., Sammon, J., Gaustad, K., Mei, F., and Longbottom, C.: Tethered Balloon System (TBS) Instrument Handbook, DOE/SC-ARM-TR-206, https://doi.org/10.2172/1415858, 2024.
Dieu Hien, V. T., Lin, C., Thanh, V. C., Kim Oanh, N. T., Thanh, B. X., Weng, C.-E., Yuan, C.-S., and Rene, E. R.: An overview of the development of vertical sampling technologies for ambient volatile organic compounds (VOCs), J. Environ. Manage., 247, 401–412, https://doi.org/10.1016/j.jenvman.2019.06.090, 2019.
Dreessen, J., Ren, X., Gardner, D., Green, K., Stratton, P., Sullivan, J. T., Delgado, R., Dickerson, R. R., Woodman, M., Berkoff, T., Gronoff, G., and Ring, A.: VOC and trace gas measurements and ozone chemistry over the Chesapeake Bay during OWLETS-2, 2018, J. Air Waste Manage., 73, 178–199, https://doi.org/10.1080/10962247.2022.2136782, 2023.
Gallego, E., Roca, F. J., Perales, J. F., Sánchez, G., and Esplugas, P.: Characterization and determination of the odorous charge in the indoor air of a waste treatment facility through the evaluation of volatile organic compounds (VOCs) using TD–GC/MS, Waste Manage., 32, 2469–2481, https://doi.org/10.1016/j.wasman.2012.07.010, 2012.
Gilman, J. B., Lerner, B. M., Kuster, W. C., Goldan, P. D., Warneke, C., Veres, P. R., Roberts, J. M., de Gouw, J. A., Burling, I. R., and Yokelson, R. J.: Biomass burning emissions and potential air quality impacts of volatile organic compounds and other trace gases from fuels common in the US, Atmos. Chem. Phys., 15, 13915–13938, https://doi.org/10.5194/acp-15-13915-2015, 2015.
Gkatzelis, G. I., Coggon, M. M., Mcdonald, B. C., Peischl, J., Aikin, K. C., Gilman, J. B., Trainer, M., and Warneke, C.: Identifying Volatile Chemical Product Tracer Compounds in U.S. Cities, Environ. Sci. Technol., 55, 188–199, https://doi.org/10.1021/acs.est.0c05467, 2021a.
Gkatzelis, G. I., Coggon, M. M., Mcdonald, B. C., Peischl, J., Gilman, J. B., Aikin, K. C., Robinson, M. A., Canonaco, F., Prevot, A. S. H., Trainer, M., and Warneke, C.: Observations Confirm that Volatile Chemical Products Are a Major Source of Petrochemical Emissions in U.S. Cities, Environ. Sci. Technol., 55, 4332–4343, https://doi.org/10.1021/acs.est.0c05471, 2021b.
Greenberg, J.: Tethered balloon measurements of biogenic VOCs in the atmospheric boundary layer, Atmos. Environ., 33, 855–867, https://doi.org/10.1016/s1352-2310(98)00302-1, 1999.
Greenberg, J. P., Guenther, A. B., Pétron, G., Wiedinmyer, C., Vega, O., Gatti, L. V., Tota, J., and Fisch, G.: Biogenic VOC emissions from forested Amazonian landscapes, Glob. Change Biol., 10, 651–662, https://doi.org/10.1111/j.1365-2486.2004.00758.x, 2004.
Guenther, A. B., Jiang, X., Heald, C. L., Sakulyanontvittaya, T., Duhl, T., Emmons, L. K., and Wang, X.: The Model of Emissions of Gases and Aerosols from Nature version 2.1 (MEGAN2.1): an extended and updated framework for modeling biogenic emissions, Geosci. Model Dev., 5, 1471–1492, https://doi.org/10.5194/gmd-5-1471-2012, 2012.
Helin, A., Hakola, H., and Hellén, H.: Optimisation of a thermal desorption–gas chromatography–mass spectrometry method for the analysis of monoterpenes, sesquiterpenes and diterpenes, Atmos. Meas. Tech., 13, 3543–3560, https://doi.org/10.5194/amt-13-3543-2020, 2020.
Helmig, D., Balsley, B., Davis, K., Kuck, L. R., Jensen, M., Bognar, J., Smith Jr, T., Arrieta, R. V., Rodriguez R., and Birks, J. W.: Vertical profiling and determination of landscape fluxes of biogenic nonmethane hydrocarbons within the planetary boundary layer in the Peruvian Amazon, J. Geophys. Res.-Atmos., 103, 25519–25532, https://doi.org/10.1029/98JD01023, 1998.
Hurley, J. F., Caceres, A., McGlynn, D. F., Tovillo, M. E., Pinar, S., Schürch, R., Onufrieva, K., and Isaacman-VanWertz, G.: Portable, low-cost samplers for distributed sampling of atmospheric gases, Atmos. Meas. Tech., 16, 4681–4692, https://doi.org/10.5194/amt-16-4681-2023, 2023.
Jardine, K. J., Jardine, A. B., Souza, V. F., Carneiro, V., Ceron, J. V., Gimenez, B. O., Soares, C. P., Durgante, F. M., Higuchi, N., Manzi, A. O., Gonçalves, J. F. C., Garcia, S., Martin, S. T., Zorzanelli, R. F., Piva, L. R., and Chambers, J. Q.: Methanol and isoprene emissions from the fast growing tropical pioneer species Vismia guianensis (Aubl.) Pers. (Hypericaceae) in the central Amazon forest, Atmos. Chem. Phys., 16, 6441–6452, https://doi.org/10.5194/acp-16-6441-2016, 2016.
Karl, T., Guenther, A., Yokelson, R. J., Greenberg, J., Potosnak, M., Blake, D. R., and Artaxo, P.: The tropical forest and fire emissions experiment: Emission, chemistry, and transport of biogenic volatile organic compounds in the lower atmosphere over Amazonia, J. Geophys. Res.-Atmos., 112, https://doi.org/10.1029/2007JD008539, 2007.
Kim, J.-C.: Factors controlling natural VOC emissions in a southeastern US pine forest, Atmos. Environ., 35, 3279–3292, https://doi.org/10.1016/S1352-2310(00)00522-7, 2001.
Leitner, S., Feichtinger, W., Mayer, S., Mayer, F., Krompetz, D., Hood-Nowotny, R., and Watzinger, A.: UAV-based sampling systems to analyse greenhouse gases and volatile organic compounds encompassing compound-specific stable isotope analysis, Atmos. Meas. Tech., 16, 513–527, https://doi.org/10.5194/amt-16-513-2023, 2023.
Li, Y., Liu, B., Ye, J., Jia, T., Khuzestani, R. B., Sun, J. Y., Cheng, X., Zheng, Y., Li, X., Wu, C., Xin, J., Wu, Z., Tomoto, M. A., Mckinney, K. A., Martin, S. T., Li, Y. J., and Chen, Q.: Unmanned Aerial Vehicle Measurements of Volatile Organic Compounds over a Subtropical Forest in China and Implications for Emission Heterogeneity, Acs Earth Space Chem, 5, 247–256, https://doi.org/10.1021/acsearthspacechem.0c00271, 2021.
Liu, Y., Seco, R., Kim, S., Guenther, A. B., Goldstein, A. H., Keutsch, F. N., Springston, S. R., Watson, T. B., Artaxo, P., Souza, R. A. F., McKinney, K. A., and Martin, S. T.: Isoprene photo-oxidation products quantify the effect of pollution on hydroxyl radicals over Amazonia, Sci. Adv., 4, eaar2547, https://doi.org/10.1126/sciadv.aar2547, 2018.
McKinney, K. A., Wang, D., Ye, J., de Fouchier, J.-B., Guimarães, P. C., Batista, C. E., Souza, R. A. F., Alves, E. G., Gu, D., Guenther, A. B., and Martin, S. T.: A sampler for atmospheric volatile organic compounds by copter unmanned aerial vehicles, Atmos. Meas. Tech., 12, 3123–3135, https://doi.org/10.5194/amt-12-3123-2019, 2019.
Müller, M., Mikoviny, T., Feil, S., Haidacher, S., Hanel, G., Hartungen, E., Jordan, A., Märk, L., Mutschlechner, P., Schottkowsky, R., Sulzer, P., Crawford, J. H., and Wisthaler, A.: A compact PTR-ToF-MS instrument for airborne measurements of volatile organic compounds at high spatiotemporal resolution, Atmos. Meas. Tech., 7, 3763–3772, https://doi.org/10.5194/amt-7-3763-2014, 2014.
Park, C., Schade, G. W., and Boedeker, I.: Characteristics of the flux of isoprene and its oxidation products in an urban area, J. Geophys. Res.-Atmos., 116, https://doi.org/10.1029/2011JD015856, 2011.
Permar, W., Wang, Q., Selimovic, V., Wielgasz, C., Yokelson, R. J., Hornbrook, R. S., Hills, A. J., Apel, E. C., Ku, I.-T., Zhou, Y., Sive, B. C., Sullivan, A. P., Collett Jr, J. L., Campos, T. L., Palm, B. B., Peng, Q., Thornton, J. A., Garofalo, L. A., Farmer, D. K., Kreidenweis, S. M., Levin, E. J. T., DeMott, P. J., Flocke, F., Fischer, E. V., and Hu, L.: Emissions of Trace Organic Gases From Western U.S. Wildfires Based on WE-CAN Aircraft Measurements, Jo. Geophys. Res.-Atmos., 126, e2020JD033838, https://doi.org/10.1029/2020JD033838, 2021.
Ribes, A., Carrera, G., Gallego, E., Roca, X., Berenguer, M. J., and Guardino, X.: Development and validation of a method for air-quality and nuisance odors monitoring of volatile organic compounds using multi-sorbent adsorption and gas chromatography/mass spectrometry thermal desorption system, J. Chromatogr. A, 1140, 44–55, https://doi.org/10.1016/j.chroma.2006.11.062, 2007.
Sasidharan, S., He, Y., Akherati, A., Li, Q., Li, W., Cocker, D., McDonald, B. C., Coggon, M. M., Seltzer, K. M., Pye, H. O. T., Pierce, J. R., and Jathar, S. H.: Secondary Organic Aerosol Formation from Volatile Chemical Product Emissions: Model Parameters and Contributions to Anthropogenic Aerosol, Environ. Sci. Technol., 57, 11891–11902, https://doi.org/10.1021/acs.est.3c00683, 2023.
Schieweck, A., Gunschera, J., Varol, D., and Salthammer, T.: Analytical procedure for the determination of very volatile organic compounds (C3–C6) in indoor air, Anal. Bioanal. Chem., 410, 3171–3183, https://doi.org/10.1007/s00216-018-1004-z, 2018.
Shrestha, S., Yoon, S., Erickson, M. H., Guo, F., Mehra, M., Bui, A. A. T., Schulze, B. C., Kotsakis, A., Daube, C., Herndon, S. C., Yacovitch, T. I., Alvarez, S., Flynn, J. H., Griffin, R. J., Cobb, G. P., Usenko, S., and Sheesley, R. J.: Traffic, transport, and vegetation drive VOC concentrations in a major urban area in Texas, Sci. Total Environ., 838, 155861, https://doi.org/10.1016/j.scitotenv.2022.155861, 2022.
Shrestha, S., Yoon, S., Alvarez, S. L., Wang, Y., Flynn, J. H., Usenko, S., and Sheesley, R. J.: Emission Ratios and Diurnal Variability of Volatile Organic Compounds and Influence of Industrial Emissions in Two Texas Cities, Atmosphere, 14, 1006, https://doi.org/10.3390/atmos14061006, 2023a.
Shrestha, S., Zhou, S., Mehra, M., Guagenti, M., Yoon, S., Alvarez, S. L., Guo, F., Chao, C.-Y., Flynn III, J. H., Wang, Y., Griffin, R. J., Usenko, S., and Sheesley, R. J.: Evaluation of aerosol- and gas-phase tracers for identification of transported biomass burning emissions in an industrially influenced location in Texas, USA, Atmos. Chem. Phys., 23, 10845–10867, https://doi.org/10.5194/acp-23-10845-2023, 2023b.
Spirig, C., Guenther, A., Greenberg, J. P., Calanca, P., and Tarvainen, V.: Tethered balloon measurements of biogenic volatile organic compounds at a Boreal forest site, Atmos. Chem. Phys., 4, 215–229, https://doi.org/10.5194/acp-4-215-2004, 2004.
Steiner, A. L.: Role of the Terrestrial Biosphere in Atmospheric Chemistry and Climate, Accounts of Chem. Res., 53, 1260–1268, 10.1021/acs.accounts.0c00116, 2020.
Ventura Diaz, P. and Yoon, S.: High-Fidelity Computational Aerodynamics of Multi-Rotor Unmanned Aerial Vehicles, 2018 AIAA Aerospace Sciences Meeting, Kissimmee, Florida, 8–12 January 2018, AIAA 2018-1266, https://doi.org/10.2514/6.2018-1266, 2018.
Walter, P., Guagenti, M, Usenko, S., Sheesley, R. J., and Flynn, J.: TRACER-Tethersonde VOC data, ARM Live Data Web Service [data set], https://doi.org/10.5439/1995524, 2025a.
Walter, P., Guagenti, M, Usenko, S., Sheesley, R. J., and Flynn, J.: TRACER-Tethersonde ozone data, ARM Live Data Web Service [data set], https://doi.org/10.5439/2007751, 2025b.
Yang, S., Li, X., Zeng, L., Yu, X., Liu, Y., Lu, S., Huang, X., Zhang, D., Xu, H., Lin, S., Liu, H., Feng, M., Song, D., Tan, Q., Cui, J., Wang, L., Chen, Y., Wang, W., Sun, H., Song, M., Kong, L., Liu, Y., Wei, L., Zhu, X., and Zhang, Y.: Development of multi-channel whole-air sampling equipment onboard an unmanned aerial vehicle for investigating volatile organic compounds' vertical distribution in the planetary boundary layer, Atmos. Meas. Tech., 16, 501–512, https://doi.org/10.5194/amt-16-501-2023, 2023.
Zhai, H., Zhou, J., Zhao, W., Du, W., Wei, N., Liu, Q., Wang, S., Zhang, J., Cui, W., Zhang, W., Lu, Y., Zhu, Z., and Liu, Y.: Development of a UAV-borne sorbent tube sampler and its application on the vertical profile measurement of volatile organic compounds, J. Environ. Sci., 152, 276–286, https://doi.org/10.1016/j.jes.2024.04.016, 2025.