the Creative Commons Attribution 4.0 License.
the Creative Commons Attribution 4.0 License.
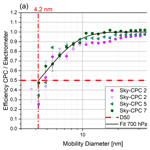
Characterisation of a self-sustained, water-based condensation particle counter for aircraft cruising pressure level operation
Oliver F. Bischof
Benedikt Fischer
Marcel Berg
Susanne Hering
Steven Spielman
Gregory Lewis
Andreas Petzold
Aerosol particle number concentration measurements are a crucial part of aerosol research. Vertical profile measurements and high-altitude/low-pressure performance of the respective instruments become more important for remote sensing validation and a vital tool for the observation of climate variables. This study tests the new, commercially available water condensation particle counter (MAGIC 210-LP) for the deployment at aircraft cruising pressure levels that the European research infrastructure IAGOS (In-service Aircraft for a Global Observing System; http://www.iagos.org, last access: 2 May 2023) is aiming for by operating measurement instrumentation onboard passenger aircraft. We conducted laboratory experiments for conditions to simulate passenger aircraft flight altitude at operation pressure. We demonstrate that this type of water condensation particle counter shows excellent agreement with a butanol-based instrument used in parallel. A Faraday cup aerosol electrometer serves as the reference instrument. Experiments are performed with test aerosol ammonium sulfate and fresh combustion soot at pressure levels ranging from 700 to 200 hPa. For soluble particles like ammonium sulfate, the 50 % detection efficiency cut-off diameter (D50) is around 5 nm and does not differ significantly for all performed experiments. For non-soluble fresh soot particles, the D50 cut-off diameter of approximately 10 nm does not vary substantially as a function of pressure, whereas the 90 % detection efficiency cut-off diameter D90 increases from 19 nm at 700 hPa to 37 nm at 200 hPa. The overall counting efficiency for particles larger than 40 nm reaches 100 % for working pressures of 200 hPa and higher.
- Article
(1835 KB) - Full-text XML
-
Supplement
(709 KB) - BibTeX
- EndNote
Condensation particle counters (CPCs) experienced a rise in use in recent years, driven by the increasing awareness of the adverse effects of particles on air quality and public health (von Schneidemesser et al., 2015). Specifically, the monitoring of atmospheric aerosol on the ground (McMurry, 2000) as well as on airborne platforms (Petzold et al., 2013) and the measurement of exhaust aerosol from various sources (Giechaskiel et al., 2009; Petzold et al., 2011; Bischof et al., 2020), indoor aerosol (Salimifard et al., 2020), and airborne viruses in the still ongoing pandemic (Somsen et al., 2020) are current key applications of condensation particles counters.
A condensation particle counter (CPC) measures the aerosol particle number concentration by activating nanometre-sized particles in a supersaturated environment and further growing them to optically detectable droplets in the lower micrometre size range. Single particles are subsequently detected and counted utilising a photodetector to measure the intensity of the scattered radiation of a laser beam. John Aitken is known for his early experiments in which he counted particles that had grown in an expansion chamber due to the supersaturation of water vapour (Aitken, 1888).
In general terms, the measurement principle of a CPC can be broken down into three steps: saturation, by which a saturated vapour of a working fluid is formed inside the CPC; supersaturation and subsequent condensation, by which vapour condenses on the particle; and detection, by which the enlarged particles scatter light when passing through a laser beam which is then counted with a photodiode – see e.g. Bischof (2022), Hinds (1999), and Cheng (2011).
Today, mainly three working fluids are in use: n-butyl alcohol (or n-butanol), water, and isopropyl alcohol (2-propanol) or isopropanol. For low-pressure applications, perfluorotributylamine can be used. For all working fluids, detection efficiency experiments have been conducted over a specific operation pressure range (e.g. Brock et al., 2000; Bundke et al., 2015; Gallar et al., 2006; Hermann et al., 2007; Williamson et al., 2018), which demonstrated the applicability of each working fluid for low-pressure operation CPCs. It should be noted that the use of both butanol and isopropanol is limited by the fact that (1) both are flammable liquids and (2) can take up water at high humidity, which influences the counting efficiency, whereas water has the advantage to avoid health and safety concerns of butanol. Disadvantageously, water has a three times higher mass diffusion coefficient than butanol, which increases the consumption of the working fluid during operation (Hering et al., 2005; Mei et al., 2021).
Global aerosol observation is targeted by the European research infrastructure IAGOS (In-service Aircraft for a Global Observing System) (Petzold et al., 2015), which aims to cover all essential climate variables of the atmosphere, including aerosol particles (Bojinski et al., 2014) by regular and global-scale measurements conducted on board of a fleet of passenger aircraft equipped with automated scientific instrumentation. The IAGOS aerosol instrument using butanol-based CPC is described in detail by Bundke et al. (2015) and provided the first results during the observation of the Raikoke volcanic ash plume by IAGOS (Osborne et al., 2022). However, because of its flammability, butanol on passenger aircraft requires special permission, which we could not attain. Instead, the application of water-based CPCs is highly advisable, mainly under consideration of flight security aspects.
This study is part of the development of a new air quality instrument for IAGOS in response to these flight safety aspects. The package consists of a modified portable optical particle spectrometer (POPS; Gao et al., 2016; originally developed by NOAA), which measures the particle size distribution in the diameter range from 125 nm to 4 µm; four cavity attenuated phase shift (CAPS; Kebabian et al., 2005, 2007; Aerodyne Research Inc., Billerica, MA, USA) instruments to measure the particle extinction coefficients at different wavelengths as well as the NO2 concentration; and finally the water-based MAGIC 210-LP CPC to measure the total particle number concentration, which is characterised in this work.
The new water-based condensation particle counter (MAGIC 210-LP; Moderated Aerosol Growth with Internal water Cycling – Low Pressure) for low-pressure applications down to 300 hPa characterised in this study was recently introduced to the market by Aerosol Dynamics Inc. and is based on the standard MAGIC CPC, which contains a pre-humidifier, where the aerosol sampling flow is guided to a continuous wet wick with different temperature zones. The humidified sample flow starts with the cold conditioner region, then the warm initiator and a cold moderator zone before finally passing the optics head (Hering et al., 2019). The MAGIC 210-LP CPC was subjected to counting efficiency experiments for a pressure range down to 200 hPa and different types of test aerosol particles representing salt particles and non-soluble particles. The conducted experiments were part of the qualification of the individual components of the new IAGOS air quality instrument.
A schematic of the experimental set-up is shown in Fig. 1. We used a nebuliser to atomise an ammonium sulfate (AS) solution to provide a steady and constant particle production in size distribution and number concentration (constant output atomiser; Model 3076, TSI Inc., Shoreview, MN, USA) (Liu and Pui, 1975; TSI Inc. Model 3076, manual). After the aerosol flow passes through a diffusion dryer tube, the relative humidity reaches levels below 5 %. The sample flow follows a charging process by passing through a radioactive Am-241 source and the classification in a monodisperse aerosol takes place by a Vienna-type differential mobility analyser (DMA; Model M-DMA 55-U, Grimm Aerosol Technik GmbH & Co. KG, Ainring, Germany). The aerosol enters the low-pressure zone by passing through a critical orifice. The aerosol is diluted in the mixing chamber with aerosol-filtered air. The pressure is controlled by a LabVIEW programme through multiple mass flow controllers with a PID (proportional integral derivative) controller approach. At 200 hPa, the measured standard deviation was less than 0.1 hPa with an integration time of 100 s. Furthermore, the relative humidity is actively controlled by adding a stable humidified air flow into the system through the mixing chamber, which is limited to approximately 30 % relative humidity. Temperature, inline pressure, and relative humidity are measured in the mixing chamber. Water vapour can be added to test particle activation growth effects for different relative humidity levels. The volume of the mixing chamber is 500 mL with a flow rate of 10 L min−1. This leads to a flushing time of roughly 3 s and an e-folding time of 1.8 s for 63 %. After passing through the mixing chamber, the aerosol flow is guided to the measuring instruments using individual isokinetic, iso-axial samplers located in the centre of the sample line. The diffusion losses are similar for all instruments. The flexible conductive sampling tubing length from the line to the instruments is set to 25 cm for instruments sampling at a flow of 0.6 L min−1 and adjusted proportionally to instruments with a different sampling flow. A Sky-CPC 5.411 (Grimm) was used as a well-characterised butanol condensation particle counter (Bundke et al., 2015). An aerosol electrometer was used as a traceable reference instrument for particle counting measurements (FCE; Model 5.705, Grimm). The instrument of interest was the newly developed Moderated Aerosol Growth with Internal Water Cycling CPC (MAGIC 210-LP; Aerosol Dynamics Inc, Berkeley CA, USA). For the fresh flame soot measurements, the nebuliser and the dehydration tube were replaced by a miniature inverted flame soot generator (Argonaut Scientific Corp., Edmonton, AB, Canada). Prior studies provide greater detail (Bundke et al., 2015; Bischof, 2022).
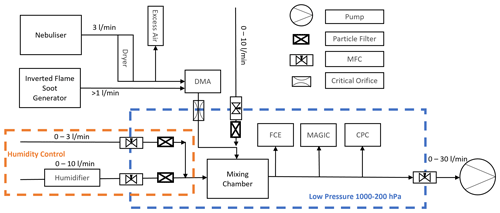
Figure 1Flow schematic of the laboratory set-up for the low-pressure characterisation with two aerosol sources. The inline pressure is controlled via mass flow controllers (MFCs), the aerosol size classification is ensured with a differential mobility analyser (DMA), and the Faraday cup electrometer (FCE) functions as a reference instrument for particle counting.
The DMA was operated stepwise for 30 s for each voltage level corresponding to different particle sizes starting at an upper limit of 140 nm and going down to 2.5 nm. This size limit is set by the fact that we used the 8.8 cm tube and a 6 L min−1 sheath flow for a high accuracy and low-mobility size half-width. To avoid transition effects and to achieve a uniform aerosol inside all measuring instruments, the first 15 s for each particle size setting of the DMA were excluded from the dataset. Earlier experiments have shown that this time is sufficient to flush the system.
The inverted flame soot generator was operated with an oxidation-air-to-propane ratio of 7.5 L min−1 air to 0.0625 L min−1 propane. This flow setting ensures stable low organic carbon soot production (Bischof et al., 2020; Kazemimanesh et al., 2019).
Data analysis procedure
A significant issue for measuring nanometre-sized particles arises from using a DMA as a size selector based on particle mobility; therefore, multiply charged particles must be accounted for when analysing electrometer counting statistics. The DMA selects particles according to their charge-to-size ratio by the DMA because their electrical mobility is identical compared to singly charged particles but are larger in size. These multiple charges lead to a notable difference in the counting rate between a condensation particle counter and an aerosol electrometer. A correction scheme was used to address multiply charged particles biasing the concentration discrepancy. The routine was first introduced by Bundke (Bundke et al., 2015; Bischof, 2022). The full data inversion is simplified and shown as a flowchart in Fig. 2. Further explanation is given in the Supplement.
An exponential function (Eq. 1) introduced by Wiedensohler et al. (1997) is used to give quantitative information about the cut-off efficiencies. Here, the revised formulation by Banse et al. (2001) provides a more quantitative description of the particle counting efficiency curves compared to the electrometer.
Here, η is the counting efficiency; Dp is the particle size; and A, B, D1, and D2 are fitting parameters of this four-parameter exponential function. The fitting parameter A is the maximum of the function and gives the value of the plateau.
Two critical variables for low-pressure measurements automatically control the MAGIC 210-LP CPC counting efficiency. The first variable is the laser power, which is adjusted to compensate for variations in droplet size as a function of the operating pressure. With decreasing pressure levels, droplet growth is affected, making the droplets smaller. The laser power automatically increases as the internal instrument pressure decreases to compensate for the smaller droplet size. The second variable is the detection threshold voltage, which is adjusted to compensate for variations in background scattered light (i.e. measured light with zero particle counts) as the laser power varies, since the background light scattering on molecules increases with increasing laser power. A detailed description is given in the Supplement. In the experiments, the MAGIC 210-LP CPC was operated with the temperature settings recommended for low pressures by the manufacturer in the operational manual. During normal (ambient; 1000 hPa) operation, the conditioner is maintained at 18 K below and the initiator at 17 K above the heat sink temperature, typically a few degrees above ambient. The moderator temperature is usually set as a function of input dew point to minimise water usage. The user has the option of changing this temperature or setting fixed temperatures. The manual for the MAGIC 210-LP states that the conditioner temperature should be kept at 2 ∘C and the moderator at 4 ∘C for low-pressure operations. The initiator is fixed at 45 ∘C to remain below the boiling point when operating at pressures as low as 150 hPa. However, these working points cannot be reached if the heat sink exceeds temperatures of 33 ∘C. During measurement, a heatwave occurred, and it became clear that the thermoelectrical devices had reached their limits. It was then observed that in case the temperatures of the conditioner and the moderator are about 3 K above their recommended values, the counting efficiency decreases by about 20 % from 100 % to 80 % overall counting efficiency at pressure levels 250 hPa and below. This limitation, however, is solvable by maintaining the ΔT between all temperature zones of the sections of the growth tube equally.
The manufacturer's settings were not optimised for operating pressure down to 200 hPa. For 250 hPa, we found that the required laser power was increased so high by the firmware to compensate for the smaller droplet sizes, thus the electronics could not compensate the baseline voltage correctly. By adjusting the values for the laser power and detector threshold, we solved this issue. Therefore, the MAGIC LP-210 is now able to operate even below 250 hPa. The new settings are applicable for the complete pressure range without change. Further explanation on this is given in the Supplement. To give an overview of the two particle types we used for the evaluation studies, the aerosol size distribution for the test aerosol is shown in Fig. 3.
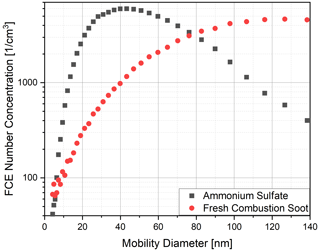
Figure 3Particle size distributions were measured by an electrometer and sized by DMA for ammonium sulfate and fresh combustion soot. For this work, the particle mobility sizes were measured to 138 nm, so the size resolution at smaller sizes is suitable for the cut-off characterisation. The full particle size distributions are available (Weber et al., 2022).
To achieve a high resolution for smaller particle sizes, we used the model M-DMA 55-U. The 8.8 cm DMA tube and a 6 L min−1 sheath flow as operational parameters determining upper and lower size limit. The upper size limit is at 138 nm mobility particle sizes. This upper size limit is sufficient to characterise the ammonium sulfate size distribution, but this size limit covers only parts of the fresh combustion soot size distribution. The ratio of the sample flow to sheath flow rate of 0.12 leads to a narrow mobility size half-width.
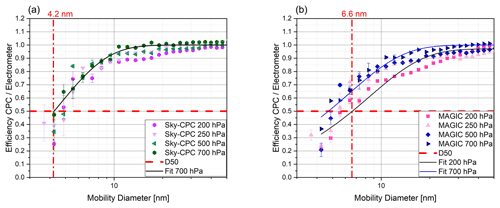
Figure 4Compilation of the efficiency ratio curves of the SKY-CPC 5.411 (SKY-CPC) (a) and the MAGIC 210-LP CPC (b) to the FCE reference – at different operation pressures as a function of the particle size using ammonium sulfate particles. The variance of the measurement is indicated by vertical bars.
The overall counting efficiency, the cut-off diameter, and the linearity of the two condensation particle counters compared to the electrometer was used as a reference instrument. This is essential for the instrument validation for IAGOS operation conditions. The measured particle concentrations were compared to the electrometer concentrations corrected for multiple-charged particles. First, we demonstrate the overall efficiency of the instrument by using ammonium sulfate as a particle type. Ammonium sulfate is a common particulate matter compound in the atmosphere. Fresh combustion soot as a second aerosol type is interesting because it may serve as a proxy for anthropogenic aerosol. In particular, the MAGIC should be able to measure non-volatile particle matter emissions from aircraft engines operating on IAGOS. Figure 4 shows the particle size-dependent counting efficiency of the SKY-CPC and the MAGIC 210-LP with respect to the multiple-charge-corrected FCE reference measurements. To offer a clear picture of the cut-off diameter, we do not show detailed data above 50 nm, since the instrument reaches a stable plateau of the counting efficiency. In Fig. 5, the scatterplot demonstrates the overall linearity between the instruments by using the number concentration distribution visible in Fig. 3.
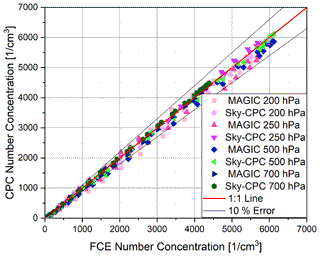
Figure 5Comparison of the counting linearity between both CPC types and the electrometer at different pressure levels for nebulised ammonium sulfate.
Using ammonium sulfate as a particle material, the instruments respond with an excellent agreement with the FCE reference instrument, with a slope of 1.0 ± 0.05 regardless of the inline pressure. The MAGIC 210-LP and the SKY-CPC scatter around the 1 : 1 line, showing counting linearity for the full spectrum of particle concentrations.
Table 1Coefficients of the exponential function of the counting efficiency curves for the SKY-CPC 5.411 (SKY-CPC) and the MAGIC 210-LP CPC and for different line pressure values and ammonium sulfate.
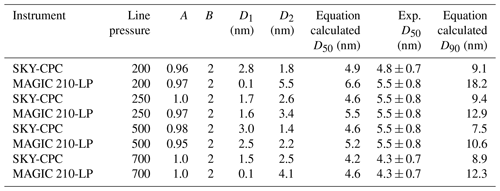
When looking deeper into the detail at small particle sizes, both CPCs show a D50 cut-off diameter of around 5 nm at all pressure levels (see Table 1). The reported D50 value is in accordance with previous measurements performed with the standard MAGIC instrument, using ammonium sulfate as aerosol material (Hering et al., 2005). When operated at reduced pressures, the SKY-CPC shows no significant change in counting efficiency behaviour. The MAGIC 210-LP counts at least 90 % of the particles compared to the electrometer for pressure levels higher than 250 hPa and particle sizes larger than 30 nm. As the operation pressure reaches 200 hPa, the counting efficiency suffers from a small drop to about 80 %, but only for particles smaller than 15 nm. The laser power and detector threshold parameters were chosen to cover all pressures down to 200 hPa.
We used combustion soot as a second particle type utilising the miniature inverted flame soot generator (Bischof et al., 2020). We used the second type to show the behaviour of an aerosol that does not dissolve in a liquid. The experimental set-up was therefore adjusted by replacing the nebuliser and its subsequent diffusion dryer with the inverted flame soot generator.
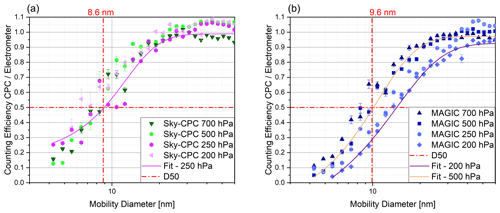
Figure 6Comparison of the efficiency ratio curves of the SKY-CPC 5.411 (SKY-CPC) (a) and the MAGIC 210-LP CPC (b) to the electrometer reference at different operating pressures as a function of the particle size using fresh combustion soot. The variance of the measurement is indicated by vertical bars.
Figures 6 and 7 show the comparison between the Grimm CPC, MAGIC 210-LP, and the electrometer for the freshly generated combustion aerosol at different operating pressure levels. The SKY-CPC and the MAGIC 210-LP show nearly identical behaviour for counting efficiencies at pressures higher than 250 hPa. For lower pressure, the SKY-CPC continues to measure with the same efficiency. As with a gradual shift with decreasing pressure to 200 hPa, the D50 cut-off of the MAGIC 210-LP increases to around 15 nm and its D90 to about 40 nm.
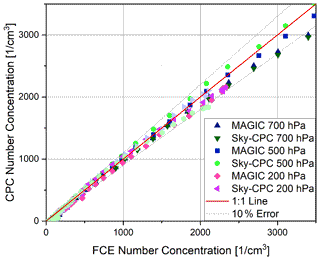
Figure 7Comparison of the counting linearity between CPC and the electrometer at different pressure levels for fresh combustion soot.
As an insoluble hydrophobic substance, fresh combustion soot is not activated for droplet formation inside a CPC as efficiently as hydrophilic substances (Petzold et al., 2005). Therefore, soot particles need to be more prominent in diameter for nucleus activation than hydrophilic particles, which explains the increase of the D50 compared to our ammonium sulfate experiments. For overall airborne measurements, it is unlikely to encounter fresh combustion soot. The only case where we have identified fresh soot emissions at these heights are areas of crossing fresh aircraft plume visible as 1–2 s peaks in the time series in heavily used flight corridor routes. Furthermore, fresh combustion soot is a good proxy for non-hydrophilic substances.
Table 2Coefficients of the exponential function of the counting efficiency curves for the SKY-CPC 5.411 (SKY-CPC) and the MAGIC 210-LP CPC for different line pressure values and fresh combustion soot.
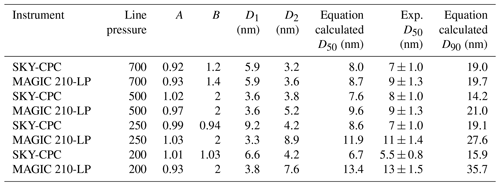
Tables 1 and 2 give an overview of the counting linearity of the CPC-type instruments with respect to the FCE reference instrument, for both aerosol types. The Pearson correlation coefficient r2 never drops below 0.99 for each measurement. Concerning the instrument linearity for soot particles, the MAGIC 210-LP and the SKY-CPC underdetermines particle number concentration compared to the FCE with increasing total number concentrations at 700 hPa. However, this effect was not observed for the other pressure ranges. The average counting efficiency for the 700 hPa trials is still within the overall uncertainty (10 %) (Petzold et al., 2011) for all counting efficiency measurements; see parameter A in Tables 1 and 2 for details.
Looking at the D50 value in Tables 1 and 2, both instruments show a cut-off diameter close to 5 nm for ammonium sulfate. This agrees with the reported detection limit for both instruments from the manufacturer and publications (Hering et al., 2014; Bischof, 2022).
The overall trend for the MAGIC 210-LP becomes apparent when looking deeper into the cut-off efficiency measurements. The difference between D50 and D90 increases with decreasing pressure, resulting in a less steep decrease in the counting efficiency towards smaller particle sizes. This feature then causes a higher uncertainty for measurements of the total number concentration of an aerosol with a strong Aitken mode, particularly for low-pressure surroundings. Whereas the D50 value does not change significantly, the D90 increases significantly. The overall difference between D50 and D90 indicates a shallow decrease in counting efficiency over a wide particle size range. Switching the particle type to soot, the lower size detection limits increase because of its less efficient activation.
Analysing the behaviour of the fitting parameter A, which represents the plateau of the fit function, and the derived parameter D50 of the fitting function in Tables 1 and 2, no clear trend is visible for the two aerosol types and instruments. The values of D50, deduced from the fitting curves, are close to 5 nm for both condensation particle counters and all pressure levels in the case of ammonium sulfate (Table 1) and fit the experimental data. For fresh combustion soot (Table 2), D50 values for the SKY-CPC instrument are slightly larger at a value of 8 nm, while for the MAGIC 210-LP, the increase in D50 compared to ammonium sulfate is more pronounced. Overall, the agreement between values derived directly from the experiment and values deduced from the fitting procedure is within the error margin of the individual mobility size.
At lower pressures, the particle counting efficiency drops for small particle sizes in the Aitken mode range and below. Because of particle line losses during sampling, the overall efficiency of the measurement system is determined by the instrumental efficiency times the inlet efficiency. Thus, inlet line loss impacts the quality of the measurements directly. In case in the foreseen application of the instrument onboard passenger aircraft equipped with IAGOS instruments using a sampling line of more than 1 m length line losses are significant: here 50 % (85 %) of 5 nm (13 nm) particles will penetrate to the instrument (at 150 hPa and 2.4 L min−1 total flow) (Bundke et al., 2015) mostly caused by diffusion losses. We show curves for the expected inlet efficiencies, including losses, in the Supplement (see Fig. S5). Yet, the overall uncertainties must be determined by modelling MAGIC 210-LP and SKY-CPC instrument responses for different aerosol size distributions, mainly with and without a nucleation mode, for IAGOS-characteristic sample line lengths.
The MAGIC CPC was recently introduced as a new water-based CPC with excellent overall performance compared to a standard butanol CPC (Hering et al., 2014, 2019). In this work, we characterised a modified “LP” (low pressure; version: MAGIC-LP 210) model of that water-based CPC design for flight altitude pressure levels as low as 200 hPa. We recommend testing each unit for low-pressure applications and adjusting the manufacturer settings when operating at pressures lower than 500 hPa if necessary. The factory settings were sufficient for operating the instrument at pressures > 500 hPa. We briefly examined the performance of five MAGIC-LP 210 units at laboratory pressure and found that the inter instrumental scatterplots follow the 1 : 1 correlation between unit. However, a side-by-side analysis of the cut-off diameter for different pressures has not been performed yet. For the units we tested, we found that laser power and detector threshold are critical for a high counting efficiency at all tested pressures. It is noted that since this study, the manufacturer has acted on the insights from this work and modified the firmware and design of the MAGIC 210-LP we tested to improve the performance at high altitudes. Automatic adjustments in the laser and detector settings with operating pressure were incorporated in the newest model MAGIC 250-LP. We will apply these new settings as part of the evaluation of the additional MAGIC-LP 210 models planned for the IAGOS payloads and recommend this for all low-pressure applications of the MAGIC 210 LP.
The MAGIC 210-LP operates at all pressure levels tested and reports reliable particle concentrations with overall detection efficiencies close to 100 % for particles larger than 40 nm. For the continuous operation on IAGOS aircraft packages, its water recycling mechanism makes the instrument attractive for long-term operation in harsh conditions with limited opportunities for instrument access and maintenance. To evaluate the instrument performance and in particular, the counting efficiency, as a function of the aerosol type and pressure, an aerosol electrometer and a butanol condensation particle counter were used as established reference instruments. For ammonium sulfate particles, the MAGIC 210-LP CPC shows excellent stability of the D50 cut-off diameter and overall linearity with an r2 of 0.99. Verified by experimental data and shown with parameters of an exponential function, the overall counting efficiency reaches 100 % for pressure levels higher than 200 hPa and particles larger than 30 nm, regardless of the particle type. However, at 200 hPa, the counting efficiency for particles smaller than 30 nm drops notably to 90 % compared to the electrometer or the butanol CPC. When the MAGIC 210-LP is exposed to a hydrophobic and insoluble particle type like fresh combustion soot, the water condensation particle counter shows similar behaviour for almost all particle sizes down to 30 nm for ambient pressure levels down to 250 hPa with linearity within 95 %. This pressure range covers the operational conditions present during IAGOS aircraft flights. For pressures down to 200 hPa, the efficiency of the MAGIC 210-LP reaches 100 % linearity towards the reference instrument for an extensive particle range. For particles smaller than 30 nm, the counting efficiency is lower than 90 %, decreasing to 70 % (60 %) for 20 nm (15 nm) particles. Because of the reduction of the counting efficiency for particles smaller than 30 nm for operational pressure levels below 250 hPa, the uncertainty of the reported number concentration is enhanced, particularly when sampling an aerosol with a strong nucleation mode, and the lower counting efficiency of the MAGIC 210-LP for smaller particle sizes results in a higher uncertainty of the total particle count.
Measurement data are available from Zenodo: https://doi.org/10.5281/zenodo.8115927 (Weber et al., 2023).
The supplement related to this article is available online at: https://doi.org/10.5194/amt-16-3505-2023-supplement.
PW performed all instrument calibrations, the instrumental set-up, and the data analysis. UB and BF designed the LabVIEW environment of the experimental set-up. MB helped during instrument preparations. StS, GL, and SH provided technical details of the instrumentation. PW, OFB, UB, and AP contributed to the paper and the interpretation of the results.
Gregory Lewis and Susanne Hering are owners and Steven Spielman is an employee of Aerosol Dynamics Inc, which developed and sells the MAGIC 210-LP. The other authors declare that they have no conflict of interest.
Publisher’s note: Copernicus Publications remains neutral with regard to jurisdictional claims in published maps and institutional affiliations.
Parts of this work were supported by the German Ministry of Research and Education in the joint research project IAGOS-D (grant agreement no. 01LK1301A) and by the HITEC Graduate School for Energy and Climate at Forschungszentrum Jülich.
This research has been supported by the Bundesministerium für Bildung und Forschung (grant no. 01LK1301A) and the Forschungszentrum Jülich (HITEC).
The article processing charges for this open-access publication were covered by the Forschungszentrum Jülich.
This paper was edited by Charles Brock and reviewed by Konrad Kandler, Christina Williamson, and one anonymous referee.
Aitken, J.: On the Number of Dust Particles in the Atmosphere, Nature, 37, 428–430, https://doi.org/10.1038/037428a0, 1888.
Banse, D. F., Esfeld, K., Hermann, M., Sierau, B., and Wiedensohler, A.: Particle counting efficiency of the TSI CPC 3762 for different operating parameters, J. Aerosol Sci., 32, 157–161, https://doi.org/10.1016/S0021-8502(00)00060-4, 2001.
Bischof, O., Weber, P., Bundke, U., Petzold, A., and Kiendler-Scharr, A.: Characterization of the Miniaturized Inverted Flame Burner as a Combustion Source to Generate a Nanoparticle Calibration Aerosol, Aerosol, Emiss. Control Sci. Technol., 6, 37–46, https://doi.org/10.1007/s40825-019-00147-w, 2020.
Bischof, O. F.: Application-Specific Calibration of Condensation Particle Counters under Low Pressure Conditions, Dissertation, Verlag des Forschungszentrums Jülich, Energie Umwelt, Band 576, ISBN 978-3-95806-629-8, 2022.
Bojinski, S., Verstraete, M., Peterson, T. C., Richter, C., Simmons, A., and Zemp, M.: The Concept of Essential Climate Variables in Support of Climate Research, Applications, and Policy, B. Am. Meteorol. Soc., 95, 1431–1443, https://doi.org/10.1175/BAMS-D-13-00047.1, 2014.
Brock, C. A., Schröder, F., Kärcher, B., Petzold, A., Busen, R., and Fiebig, M.: Ultrafine particle size distributions measured in aircraft exhaust plumes, J. Geophys. Res.-Atmos., 105, 26555–26567, https://doi.org/10.1029/2000JD900360, 2000.
Bundke, U., Berg, M., Houben, N., Ibrahim, A., Fiebig, M., Tettich, F., Klaus, C., Franke, H., and Petzold, A.: The IAGOS-CORE aerosol package: instrument design, operation and performance for continuous measurement aboard in-service aircraft, Tellus B, 67, 286–302, https://doi.org/10.3402/tellusb.v67.28339, 2015.
Cheng, Y. S.: Condensation Particle Counters, in: Aerosol Measurements, edited by: Kulkarni, P., Baron, P. A., and Willeke, K., John Wiley & Sons, Inc, New Jersey, 381–392, ISBN 978-0-470-38741-2, 2011.
Gallar, C., Brock, C. A., Jimenez, J. L., and Simons, C.: A Variable Supersaturation Condensation Particle Sizer, Aerosol Sci. Tech., 40, 431–436, https://doi.org/10.1080/02786820600643339, 2006.
Gao, R. S., Telg, H., McLaughlin, R. J., Ciciora, S. J., Watts, L. A., Richardson, M. S., Schwarz, J. P., Perring, A. E., Thornberry, T. D., Rollins, A. W., Markovic, M. Z., Bates, T. S., Johnson, J. E., and Fahey, D. W.: A light-weight, high-sensitivity particle spectrometer for PM2.5 aerosol measurements, Aerosol Sci. Tech., 50, 88–99, https://doi.org/10.1080/02786826.2015.1131809, 2016.
Giechaskiel, B., Wang, X., Horn, H. G., Spielvogel, J., Gerhart, C., Southgate, J., Jing, L., Kasper, M., Drossinos, Y., and Krasenbrink, A.: Calibration of Condensation Particle Counters for Legislated Vehicle Number Emission Measurements, Aerosol Sci. Tech., 43, 1164–1173, https://doi.org/10.1080/02786820903242029, 2009.
Hering, S. V., Stolzenburg, M. R., Quant, F. R., Oberreit, D. R., and Keady, P. B.: A Laminar-Flow, Water-Based Condensation Particle Counter (WCPC), Aerosol Sci. Tech., 39, 659–672, https://doi.org/10.1080/02786820500182123, 2005.
Hering, S. V., Spielman, S. R., and Lewis, G. S.: Moderated, Water-Based, Condensational Particle Growth in a Laminar Flow, Aerosol Sci. Tech., 48, 401–408, https://doi.org/10.1080/02786826.2014.881460, 2014.
Hering, S. V., Lewis, G. S., Spielman, S. R., and Eiguren-Fernandez, A.: A MAGIC concept for self-sustained, water-based, ultrafine particle counting, Aerosol Sci. Tech., 53, 63–72, https://doi.org/10.1080/02786826.2018.1538549, 2019.
Hermann, M., Wehner, B., Bischof, O. F., Han, H.-S., Krinke, T., Liu, W. S., Zerrath, A. F., and Wiedensohler, A.: Particle counting efficiencies of new TSI condensation particle counters, J. Aerosol Sci., 38, 674–682, 2007.
Hinds, W. C.: Aerosol Technology: Properties, Behavior, and Measurement of Airborne Particles, Wiley, ISBN 978-1-119-49404-1, 1999.
Kazemimanesh, M., Moallemi, A., Thomson, K., Smallwood, G., Lobo, P., and Olfert, J.: A novel miniature inverted-flame burner for the generation of soot nanoparticles, Aerosol Sci. Tech., 53, 184–195, https://doi.org/10.1080/02786826.2018.1556774, 2019.
Kebabian, P. L., Herndon, S. C., and Freedman, A.: Detection of nitrogen dioxide by cavity attenuated phase shift spectroscopy, Anal. Chem., 77, 724–728, https://doi.org/10.1021/ac048715y, 2005.
Kebabian, P. L., Robinson, W. A., and Freedman, A.: Optical extinction monitor using cw cavity enhanced detection, Rev. Sci. Instrum., 78, 063102, https://doi.org/10.1063/1.2744223, 2007.
Liu, B. Y. H. and Pui, D. Y. H.: On the performance of the electrical aerosol analyzer, J. Aerosol Sci., 6, 249–264, https://doi.org/10.1016/0021-8502(75)90093-2, 1975.
McMurry, P. H.: A review of atmospheric aerosol measurements, Atmos. Environ., 34, 1959–1999, https://doi.org/10.1016/s1352-2310(99)00455-0, 2000.
Mei, F., Spielman, S., Hering, S., Wang, J., Pekour, M. S., Lewis, G., Schmid, B., Tomlinson, J., and Havlicek, M.: Simulation-aided characterization of a versatile water-based condensation particle counter for atmospheric airborne research, Atmos. Meas. Tech., 14, 7329–7340, https://doi.org/10.5194/amt-14-7329-2021, 2021.
Osborne, M. J., de Leeuw, J., Witham, C., Schmidt, A., Beckett, F., Kristiansen, N., Buxmann, J., Saint, C., Welton, E. J., Fochesatto, J., Gomes, A. R., Bundke, U., Petzold, A., Marenco, F., and Haywood, J.: The 2019 Raikoke volcanic eruption – Part 2: Particle-phase dispersion and concurrent wildfire smoke emissions, Atmos. Chem. Phys., 22, 2975–2997, https://doi.org/10.5194/acp-22-2975-2022, 2022.
Petzold, A., Gysel, M., Vancassel, X., Hitzenberger, R., Puxbaum, H., Vrochticky, S., Weingartner, E., Baltensperger, U., and Mirabel, P.: On the effects of organic matter and sulphur-containing compounds on the CCN activation of combustion particles, Atmos. Chem. Phys., 5, 3187–3203, https://doi.org/10.5194/acp-5-3187-2005, 2005.
Petzold, A., Marsh, R., Johnson, M., Miller, M., Sevcenco, Y., Delhaye, D., Ibrahim, A., Williams, P., Bauer, H., Crayford, A., Bachalo, W. D., and Raper, D.: Evaluation of methods for measuring particulate matter emissions from gas turbines, Environ. Sci. Technol., 45, 3562–3568, https://doi.org/10.1021/es103969v, 2011.
Petzold, A., Formenti, P., Baumgardner, D., Bundke, U., Coe, H., Curtius, J., DeMott, P. J., Flagan, R. C., Fiebig, M., Hudson, J. G., McQuaid, J., Minikin, A., Roberts, G. C., and Wang, J.: In Situ Measurements of Aerosol Particles, in: Airborne Measurements for Environmental Research, Wiley-VCH Verlag GmbH & Co. KGaA, 157–223, https://doi.org/10.1002/9783527653218.ch4, 2013.
Petzold, A., Thouret, V., Gerbig, C., Zahn, A., Brenninkmeijer, C. A. M., Gallagher, M., Hermann, M., Pontaud, M., Ziereis, H., Boulanger, D., Marshall, J., Nédélec, P., Smit, H. G. J., Friess, U., Flaud, J.-M., Wahner, A., Cammas, J.-P., Volz-Thomas, A., and IAGOS TEAM: Global-scale atmosphere monitoring by in-service aircraft – current achievements and future prospects of the European Research Infrastructure IAGOS, Tellus B, 67, 28452, https://doi.org/10.3402/tellusb.v67.28452, 2015.
Salimifard, P., Rim, D., and Freihaut, J. D.: Evaluation of low-cost optical particle counters for monitoring individual indoor aerosol sources, Aerosol Sci. Tech., 54, 217–231, https://doi.org/10.1080/02786826.2019.1697423, 2020.
Somsen, G. A., van Rijn, C. J. M., Kooij, S., Bem, R. A., and Bonn, D.: Measurement of small droplet aerosol concentrations in public spaces using handheld particle counters, Phys. Fluids, 32, 121707, https://doi.org/10.1063/5.0035701, 2020.
von Schneidemesser, E., Monks, P. S., Allan, J. D., Bruhwiler, L., Forster, P., Fowler, D., Lauer, A., Morgan, W. T., Paasonen, P., Righi, M., Sindelarova, K., and Sutton, M. A.: Chemistry and the Linkages between Air Quality and Climate Change, Chem. Rev., 115, 3856–3897, https://doi.org/10.1021/acs.chemrev.5b00089, 2015.
Weber, P., Petzold, A., Bischof, O. F., Fischer, B., Berg, M., Freedman, A., Onasch, T. B., and Bundke, U.: Relative errors in derived multi-wavelength intensive aerosol optical properties using cavity attenuated phase shift single-scattering albedo monitors, a nephelometer, and tricolour absorption photometer measurements, Atmos. Meas. Tech., 15, 3279–3296, https://doi.org/10.5194/amt-15-3279-2022, 2022.
Weber, P., Bischof, O., Fischer, B., Berg, M., Hering, S., Spielman, S., Lewis, G., Petzold, A., and Bundke, U.: Measurement Data of Characterisation of a self-sustained, water-based condensation particle counter for aircraft cruising pressure level operation, Zenodo [data set], https://doi.org/10.5281/zenodo.8115927, 2023.
Wiedensohler, A., Orsini, D., Covert, D. S., Coffmann, D., Cantrell, W., Havlicek, M., Brechtel, F. J., Russell, L. M., Weber, R. J., Gras, J., Hudson, J. G., and Litchy, M.: Intercomparison Study of the Size-Dependent Counting Efficiency of 26 Condensation Particle Counters, Aerosol Sci. Tech., 27, 224–242, https://doi.org/10.1080/02786829708965469, 1997.
Williamson, C., Kupc, A., Wilson, J., Gesler, D. W., Reeves, J. M., Erdesz, F., McLaughlin, R., and Brock, C. A.: Fast time response measurements of particle size distributions in the 3–60 nm size range with the nucleation mode aerosol size spectrometer, Atmos. Meas. Tech., 11, 3491–3509, https://doi.org/10.5194/amt-11-3491-2018, 2018.