the Creative Commons Attribution 4.0 License.
the Creative Commons Attribution 4.0 License.
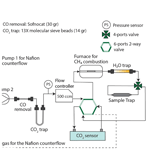
A new portable sampler of atmospheric methane for radiocarbon measurements
Lukas Wacker
Negar Haghipour
Philip Gautschi
Thomas Laemmel
Sönke Szidat
Heather Graven
Radiocarbon (14C) is an optimal tracer of methane emissions, as 14C measurements enable distinguishing between fossil methane and biogenic methane (CH4). However, 14C measurements in atmospheric methane are still rare, mainly because of the technical challenge of collecting enough carbon for 14C analysis from ambient air samples. In this study, we address this challenge by advancing the system in Zazzeri et al. (2021) into a much more compact and portable sampler and by coupling the sampler with the MICADAS (MIni CArbon DAting System) accelerator mass spectrometry (AMS) system at ETH Zurich, using a gas interface.
Here, we present the new sampler setup, the assessment of the system contamination and a first inter-laboratory comparison with LARA (Laboratory for the Analysis of Radiocarbon with AMS) at the University of Bern.
With our sampling line, we achieved a very low blank, 0.7 µgC compared to 5.5 µgC in Zazzeri et al. (2021), and a sample precision of 0.9 %, comparable with other measurement techniques for 14CH4, while reducing the sample size to 60 L of air. We show that this technique, with further improvements, will enable routine 14CH4 measurements in the field for an improved understanding of CH4 sources.
- Article
(1286 KB) - Full-text XML
- BibTeX
- EndNote
Understanding the methane (CH4) budget and identifying methane sources have become a priority to tackle global warming, as methane is the second-most-important anthropogenic greenhouse gas after carbon dioxide (CO2) and because the dynamics that led to the CH4 increase in the last decade have not been fully unravelled. Tracing CH4 sources and monitoring mitigation strategies are urgently needed.
14C measurements of atmospheric methane can advance our knowledge of methane production processes by differentiating fossil vs. biogenic sources. This is because fossil CH4 is depleted in 14C and, when emitted into the atmosphere, exerts a dilution effect on the 14C in the atmosphere that can be quantified. However, this research field is still underexplored, as 14C measurements of atmospheric methane are challenging.
One of the main challenges is sampling enough air for 14C analysis via accelerator mass spectrometry (AMS), as the atmospheric methane concentration is low (∼ 2 ppm). Here, we build on recent advances that have been made in the analysis of 14C in atmospheric methane. Traditionally, air was collected in pressurized cylinders using high-pressure pumps, followed by an extraction procedure in the laboratory (Eisma et al., 1995; Townsend-Small et al., 2012). Zazzeri et al. (2021) developed a new technique that separates methane carbon from ambient air while sampling, simplifying the transportation of collected samples in a small trap and minimizing the laboratory processing needed. The F14C measurement precision achieved is between 0.5 % and 1.2 %, comparable to the best precisions of alternative but more lab-intensive techniques. The laboratory-based system developed by Zazzeri et al. (2021) was applied in the quantification of fossil and biogenic proportions of CH4 in London (Zazzeri et al., 2023). A portable system using a similar technique was demonstrated by Palonen et al. (2017), but in that study they only used samples with enriched methane concentrations of > 100 ppm, e.g. for CH4 emissions from wetlands. Another promising recently developed technique applies chromatographic separation of CH4 from air as it requires only 60 L of atmospheric air to be sampled in a bag (Espic et al., 2019), still achieving precisions of 1.2 %.
In this study, we advance the sensitive though simple methane sampling system in Zazzeri et al. (2021) with the portability of the system by Palonen et al. (2017), requiring as little air as demonstrated by Espic et al. (2019). The result is a compact and portable system that can be deployed in field campaigns. We present the technology advancement and the assessment of the system efficiency by quantifying the amount of extraneous carbon introduced during sample preparation and ultimately the measurement precision to be achieved. We demonstrate the method by comparing 14C measurements made by the new portable system at the Laboratory of Ion beam Physics (LIP) at ETH Zurich and by the system using bag sampling and chromatographic separation at the Laboratory for the Analysis of Radiocarbon with AMS (LARA) at the University of Bern.
2.1 The sampling setup
The sampling system is based on four main steps as in Zazzeri et al. (2021): (1) H2O removal with a Nafion dryer, (2) CO and CO2 removal, (3) combustion of CH4 to CO2, and (4) adsorption of the combustion-derived CO2 onto a molecular sieve sample trap. Figure 1 shows the system schematic. Ambient air is sampled through a Nafion dryer (Perma Pure gas dryer, PD-50-24) at up to 500 cm3 min−1 with a KNF membrane pump (“Pump 2” in Fig. 1), controlled by a mass flow controller. The Nafion filter enables reduction of the water content to levels of 0.01 % (Zazzeri et al., 2021). Downstream of the pump, CO is oxidized to CO2 using the Sofnocat® catalyst before all CO2 (from ambient air and from oxidation of CO) is removed by a trap containing 14 g of 13X molecular sieve in 1 mm pellets. This amount of molecular sieve has been found to be sufficient to trap atmospheric CO2 in ∼ 300 L of air (see Fig. A1). After collection of the three samples, this trap is disconnected from the system via two Swagelok ball valves and then removed and regenerated by heating at 500 °C with a high-purity nitrogen back-flush step for at least 3 h, in a similar manner as in Zazzeri et al. (2021).
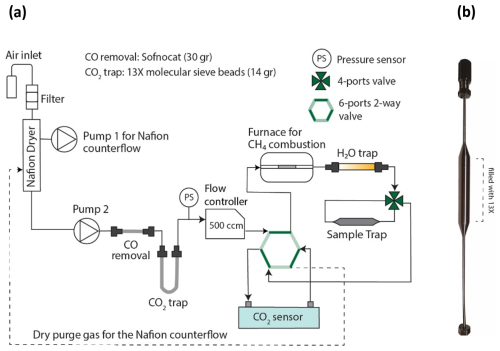
Figure 1(a) Schematic of the sampling system. First, the filtered air is dried with a Nafion dryer; then, any CO2 from ambient air and from oxidation of CO is removed by a trap. The CO2 derived from the combustion of CH4 is collected onto a final sample trap. Dark green lines in the 6-port valve indicate the active flow direction. In the indicated configuration, the CO2 sensor measures the CO2 level after the sample trap, enabling us to check for the trap breakthrough. In the alternative configuration, complete CO2 removal prior to CH4 oxidation can be checked. (b) Sample trap filled with 0.250 g of 13X.
After the sample air passes through the CO2 trap, CH4 is combusted at 800 °C in a small furnace comprising a 22 cm long quartz tube with 1 g of platinized quartz wool (Sigma-Aldrich) acting as catalyst (Petrenko et al., 2008). The H2O derived from the CH4 oxidation is trapped onto a magnesium perchlorate trap (“H2O trap” in Fig. 1), while the CO2 derived from combustion of CH4 is collected on the sample trap (13X, 45–60 mesh) for subsequent 14C measurement. A non-dispersive infrared CO2 sensor (NDIR FLOWEVO from SmartGas) monitors both the completeness of CO2 removal from air prior to methane combustion and the completeness of CO2 collection on the sample trap. If regularly calibrated and run at constant temperature and pressure, the sensor can measure CO2 concentrations in a range of 0 to 100 ppm with a precision of ±1 ppm. The sample trap, minimized in size for low cross contamination, can be cooled with Peltier coolers to maximize trapping efficiency and avoid sample loss.
The whole system runs either on 115/230 V AC or 48 V DC provided by a battery pack. Two 72 V 30 Ah (2160 Wh) lithium batteries are sufficient to run the sampling system for 10 h.
2.2 Sample trap and cooling system
The sample trap consists of 0.250 g of 13X 45–60 mesh molecular sieve packed in a 4 cm long 1/4 in. o.d. stainless-steel tube. The trap tube is welded to stainless-steel capillary tubing and attached to a VICI 4-port valve which can be disconnected from the sampling system (Fig. 1b) in order to release the sample for 14C analysis in the AMS. Before its first use, the sample trap is heated gradually to 650 °C in a customized oven while flushing with high-purity nitrogen. The NDIR FLOWEVO CO2 sensor is used to check when the trap is not releasing CO2 anymore and does not contain any residual carbon, typically after 1 h. During sampling, the sample trap is cooled down to −10 °C using two Peltier elements (part (6) in Fig. 2). This maximizes the trapping efficiency such that 0.250 g of 13X can adsorb ∼ 60 µgC (methane carbon from ∼ 60 L of ambient air at 2 ppm), before the CO2 breakthrough happens. The adsorption capacity can be enhanced by lowering the temperature even further.
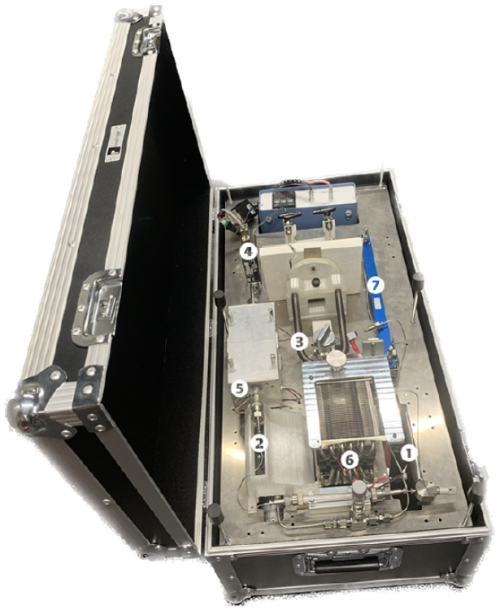
Figure 2Setup of the atmospheric methane sampling device. The whole system fits well into a box of 80 × 40 × 30 cm. It runs either on 115/230 V AC or 48 V DC provided by a battery pack. Major parts of the system are (1) the Nafion dryer (mostly hidden underneath), (2) pumps (partially hidden), (3) CO2 trap, (4) flow controller, (5) furnace for CH4 combustion, (6) sample trap with Peltier coolers, and (7) CO2 sensor.
After sampling, the sample trap is disconnected from the system and heated at 450 °C for 10 min for sample desorption using the TSE (Tube Sealing Equipment) system (https://www.ionplus.ch/tse, last access: 8 January 2025), which enables us to measure the pressure of the desorbed gases and to quantify the amount of the CO2 released. The desorbed CO2 is cryogenically sealed into a glass ampule to be used in the gas interface system of the MIni CArbon DAting System (MICADAS) (Wacker et al., 2013).
Before the next sample is collected, the sample trap is cleaned of remaining CO2 by flushing with high-purity nitrogen while heating at 550 °C for 30 min. Such a long procedure compared to other cleaning processes for other applications is only precautionary; a shorter procedure might be sufficient to remove any residual carbon from previous sampling.
2.3 AMS analysis
The sample is measured with the MICADAS accelerator mass spectrometry facilities for radiocarbon measurements in the Laboratory of Ion Beam Physics, ETH (Wacker et al., 2010). The 14C analysis using the gas interface of the MICADAS takes about 20 min and achieves a measurement precision of less than 1 % for modern samples (Wacker et al., 2013) for a sample containing 20 µg of carbon. Precisions down to 0.5 % can be achieved when measurements are repeated on ≥ 50 µg of carbon (Fahrni et al., 2013).
The combusted NOX standard (SRM-4990C, Mann, 1983; Wacker et al., 2019) and the 14C-free CO2 premixed with helium in gas bottles were measured for standard normalization or blank correction. Measured data (Zazzeri, 2025) were evaluated with the BATS computer program, where the samples were fractionation-corrected, blank-subtracted and normalized with the NOX standard (Wacker et al., 2010) to obtain F14C values (Reimer et al., 2004).
2.4 Characterization of the extraneous contaminant carbon within the sampler
Extraneous contaminant carbon in the sample trap after a sample collection might derive from intrusion of lab air into the system, from incomplete removal of atmospheric CO2, from residual carbon on the sample trap prior to sampling or from impurities within the combustion column.
To check for and quantify the contaminant carbon, we collected CH4 samples of different sizes, and we followed the relationship between the measured fraction modern (F14C) vs. the sample masses given by the mass balance in Eq. (1):
where “meas.” indicates the measured value, F14Ctrue indicates the F14C value of the sampled air, µCadd indicates the carbon added into the system and F14Cadd indicates its F14C value. If assuming a constant contamination, the contaminant carbon added to the system is given by the µCadd value that produces the best fitting curve through the F14Cmeas. values plotted against the measured sample masses (µCmeas.). We assess the goodness of fit using reduced chi-squared statistics (i.e. “curve_fit” function from the “scipi.optimize” Python package).
To quantify the modern contaminant carbon, we collected seven samples from 10 to 70 µgC from a 2 ppm mixture of fossil methane and synthetic air with no CO2, CO or hydrocarbons (Fossil Ref).
To check for any fossil contaminant, we collected seven samples, from 10 to 75 µgC, from a cylinder of pressurized ambient air (Ref 1), with a CH4 mole fraction of 2040 ppb. Note that in this case, the F14C of the reference gas (F14Ctrue) is unknown. The amount of fossil contaminant carbon and the F14C value of the reference gas are calculated by tweaking µgCadd and F14Ctrue in Eq. (1) to produce the best fitting curve.
In order to verify the source of the contaminant carbon, we collected five blanks. Three blanks were collected by running the system with lab air and without combustion for 3 h. This enabled verification of any contaminant carbon deriving from atmospheric CO2 that was not trapped in the CO2 trap and from residual carbon in the sample trap. Two samples were collected by flushing the system with nitrogen and with the combustion furnace at 800 °C to verify that additional carbon was not produced within the combustion process. No carbon was extracted from these five blanks.
2.5 Comparison with chromatographic extraction procedure
Three samples transferred in sampling bags from the cylinder of pressurized ambient air Ref 1 were extracted at LARA, University of Bern, using 60 L of air and following the chromatographic extraction procedure in Espic et al. (2019). CO2 derived from the sample extraction in Bern was measured using the gas interface system of the MICADAS AMS system at ETH, in the same manner as the samples collected with our portable sampler.
Table 1 shows the F14C values and masses of the samples collected.
Table 1Mass, F14C values and uncertainty of samples collected. Modern samples were collected from Ref 1, fossil samples were collected from Fossil Ref and “Bern” represents samples extracted following the chromatographic procedure at LARA.
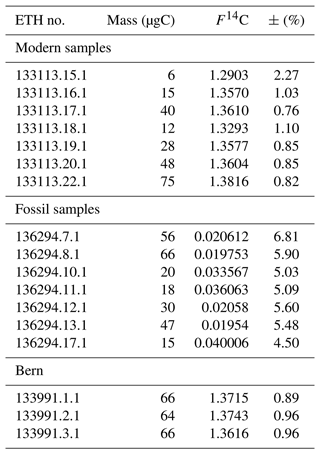
Assuming a F14Ctrue value for Fossil Ref of 0.01 and a F14C value of the modern contaminant (F14Cadd) of 1, the best fitting curve through the Fossil Ref samples indicates a constant level of modern contamination (µgCadd) of 0.5 ± 0.1 µgC. Larger samples (> 50 µgC) show an offset that can be explained with a size-dependent contamination, an additional 0.1 µgC every 10 µgC collected, which we can correct for (Fig. 3).
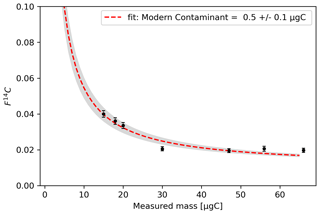
Figure 3F14C values against the measured mass of the samples collected from the Fossil Ref. The grey bands represent the 1σ uncertainty bar on the curve fit.
The data collected from Ref 1 best fit onto a curve with an F14C value of 1.38 ± 0.01 for the reference gas. However, by considering only the quantified modern contamination of 0.5 µgC, we do not achieve the best fitting curve, and we need to add approximately 0.2 ± 0.1 µg of contaminant fossil carbon. Zazzeri et al. (2021) indicated that some fossil carbon might be produced within the combustion furnace; therefore, it is likely that even with our setup the combustion process led to the production of some fossil carbon.
Ref 1 samples extracted in Bern, blue markers in Fig. 4 (not included for determining the constant contamination), agree well with the F14C values for Ref 1 samples with the same mass (60 µgC) extracted at ETH, indicating that the two extraction methods are comparable. Samples of 60 µgC are equivalent to 2 or 3 h of sampling of ambient air with our portable system at 500 sccm or 60 L of ambient air with the extraction line in Bern.
In order to make the sampler portable, we have reduced the size of the sampler components compared to the system in Zazzeri et al. (2021). The main changes include the following:
-
a smaller CO2 trap placed before the combustion furnace, with 14 g against 60 g of molecular sieve; its adsorption capacity is demonstrated by the very low modern blank, which indicates that all the ambient CO2 is captured while sampling;
-
a new design of the sample trap, with 0.250 g of molecular sieve against 1 g, accommodated in a 4 cm long tube and connected to a single 4-port VICI valve; collection of 60 µgC (2 or3 h of sampling at 500 sccm) has been achieved by cooling down the sample trap using two Peltier elements;
-
a smaller combustion furnace built at the Laboratory of Ion Beam Physics;
-
connections and tubing of 1/8 in. size instead of 1/4 in.
All these modifications led to an important reduction in the level of constant modern contamination, from 5.5 ± 1.1 µgC in Zazzeri et al. (2021) down to 0.5 ± 0.1 µgC. According to the F14C measurements of our modern reference cylinder (Ref 1), we have an extra 0.2 ± 0.1 µgC of fossil contamination, leading to 0.70 ± 0.14 µgC total amount of contaminant carbon with an averaged F14C value of 0.71. We also found a size-dependent contamination of 1 %, which can be explained either with a tiny leak within the sampler or with some outgassing.
To further test the system contamination and demonstrate full separation of CH4 and CO2, more gas mixtures could be used for F14C measurements. For example, the system could be run using a mixture made of 2 ppm of fossil CH4 and 400 ppm of CO2 from combustion of OXII (oxalic acid II), diluted in N2 or He.
The overall uncertainty for individual samples of 60 µgC, calculated by propagating the error from counting statistics and background uncertainty, is 0.9 %, comparable with other measurement techniques for 14CH4, demonstrating that a larger sample, and therefore a longer sampling time, is not needed.
The main benefit of a portable system that needs only 60 L of air for one sample is the important time saving both in the field and in the laboratory. The sample processing time in the laboratory has been reduced significantly, and so has the likelihood of contamination or mistakes by the operator. The system, given its small size, could be placed in a vehicle, enabling sampling in a source area (such as a landfill site or an urban environment) or performing a mapping of isotopic signatures in a region.
We have advanced the CH4 sampling system from Zazzeri et al. (2021) to a portable system that can be used in field campaigns while also reducing the contamination in the system. Further improvements could be made to automate the system so that the valve and pump switching and the flow rate are computer-controlled, making the whole sampling procedure more consistent. More samples could be collected in parallel and at the same time. In addition, the CO2 desorbed from the sample trap is presently cryogenically trapped in glass ampules sealed for offline 14C measurements, but a direct coupling of the zeolite trap to the gas interface (Wacker et al., 2013) connected to the MICADAS AMS system could be implemented, avoiding the additional step of using glass ampules.
Full assessment of the fossil carbon contamination in 14CH4 measurements is still challenging, because there is no modern reference material available for CH4. The production of a modern CH4 standard for 14C analysis, followed by an inter-laboratory comparison, should be pursued.
Overall, the combination of a selective and field deployable CH4 sampler and sensitive AMS analysis provides a unique technology that can expand the use of 14CH4 measurements.
The code for figures production and quantification of the contaminant carbon can be found at https://doi.org/10.5281/zenodo.14620922 (Zazzeri, 2025).
The data set including the F14C measurements can be found at https://doi.org/10.5281/zenodo.14620922 (Zazzeri, 2025).
GZ contributed to the conceptualization, formal analysis, investigation, methodology, visualization, writing and funding acquisition. LW contributed to the conceptualization, methodology and supervision. NH, PG and TL contributed to the investigation, resources and writing – review and editing, SS contributed to the resources and writing – review and editing, and HG contributed to the conceptualization and writing – review and editing.
The contact author has declared that none of the authors has any competing interests.
Publisher’s note: Copernicus Publications remains neutral with regard to jurisdictional claims made in the text, published maps, institutional affiliations, or any other geographical representation in this paper. While Copernicus Publications makes every effort to include appropriate place names, the final responsibility lies with the authors.
We are grateful for the support of the Horizon 2020 Framework Program and the work of all the engineers and trainees at the Laboratory of Ion Beam Physics that helped in the development and construction of the sampling system.
This research has been supported by the EU Horizon 2020 framework programme (call: H2020-MSCA-IF-2020, project: 101026926 – FORM) and by the Laboratory of Ion Beam Physics.
This paper was edited by Huilin Chen and reviewed by two anonymous referees.
Eisma, R., Vermeulen, A. T., and Van Der Borg, K.: 14CH4 emissions from nuclear power plants in northwestern Europe, Radiocarbon, 37, 475–483, https://doi.org/10.1017/S0033822200030952, 1995.
Espic, C., Liechti, M., Battaglia, M., Paul, D., Röckmann, T., and Szidat, S.: Compound-specific radiocarbon analysis of atmospheric methane: a new preconcentration and purification setup, Radiocarbon, 61, 1461–1476, https://doi.org/10.1017/RDC.2019.76, 2019.
Fahrni, S. M., Wacker, L., Synal, H. A., and Szidat, S.: Improving a gas ion source for 14C AMS, Nuclear Instruments and Methods in Physics Research Section B: Beam Interactions with Materials and Atoms, 294, 320–327, 2013.
Mann, W. B.: An International Reference Material for Radiocarbon Dating, Radiocarbon, 25, 519–527, 1983.
Palonen, V., Uusitalo, J., Seppälä, E., and Oinonen, M.: A portable methane sampling system for radiocarbon-based bioportion measurements and environmental CH4 sourcing studies, Rev. Sci. Instrum., 88, 075102 , https://doi.org/10.1063/1.4993920, 2017.
Petrenko, V. V., Smith, A. M., Brailsford, G., Riedel, K., Hua, Q., Lowe, D., Severinghaus, J. P., Levchenko, V., Bromley, T., Moss, R., Mühle, J., and Brook, E. J.: A New Method for Analyzing 14C of Methane in Ancient Air Extracted from Glacial Ice, Radiocarbon, 50, 53–73, https://doi.org/10.1017/S0033822200043368, 2008.
Reimer, P. J., Brown, T. A., and Reimer, R. W.: Discussion: reporting and calibration of post-bomb 14C data, Radiocarbon, 46, 1299–1304, 2004.
Townsend-Small, A., Tyler, S. C., Pataki, D. E., Xu, X., and Christensen, L. E.: Isotopic measurements of atmospheric methane in Los Angeles, California, USA: Influence of “fugitive” fossil fuel emissions, J. Geophys. Res.-Atmos., 117, D07308, https://doi.org/10.1029/2011JD016826, 2012.
Wacker, L., Bonani, G., Friedrich, M., Hajdas, I., Kromer, B., Němec, M., Ruff, M., Suter, M., Synal, H. A., and Vockenhuber, C.: MICADAS: routine and high-precision radiocarbon dating, Radiocarbon, 52, 252–262, 2010.
Wacker, L., Lippold, J., Molnár, M. and Schulz, H.: Towards radiocarbon dating of single foraminifera with a gas ion source. Nuclear Instruments and Methods in Physics Research Section B: Beam Interactions with Materials and Atoms, 294, 307–310, https://doi.org/10.1016/j.nimb.2012.08.038, 2013.
Wacker, L., Bollhalder, S., Sookdeo, A., and Synal, H. A.: Re-evaluation of the New Oxalic Acid standard with AMS. Nuclear Instruments and Methods in Physics Research Section B: Beam Interactions with Materials and Atoms, 455, 178–180, https://doi.org/10.1016/j.nimb.2018.12.035, 2019.
Zazzeri, G.: F14C measurements and python codes for quantification of contamination, Zenodo [data set/code], https://doi.org/10.5281/zenodo.14620922, 2025.
Zazzeri, G., Xu, X., and Graven, H.: Efficient sampling of atmospheric methane for radiocarbon analysis and quantification of fossil methane. Environ. Sci. Technol., 55, 8535–8541, https://doi.org/10.1021/acs.est.0c03300, 2021.
Zazzeri, G., Graven, H., Xu, X., Saboya, E., Blyth, L., Manning, A.J., Chawner, H., Wu, D., and Hammer, S.: Radiocarbon measurements reveal underestimated fossil CH4 and CO2 emissions in London, Geophys. Res. Lett., 50, e2023GL103834, https://doi.org/10.1029/2023GL103834, 2023.