the Creative Commons Attribution 4.0 License.
the Creative Commons Attribution 4.0 License.
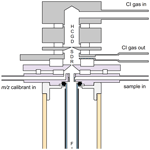
On the development of a new prototype PTR-ToF-MS instrument and its application to the detection of atmospheric amines
Alexander Håland
Tomáš Mikoviny
Elisabeth Emilie Syse
Armin Wisthaler
We herein report on the development of a new prototype PTR-ToF-MS (proton-transfer-reaction time-of-flight mass spectrometry) instrument that combines a hollow cathode glow discharge (HCGD) ion source with a focusing ion–molecule reactor (FIMR), which consists of a resistive glass drift tube surrounded by quadrupole rods. The new instrument configuration hybridizes the two main current commercial PTR-ToF-MS instrument designs. We provide a detailed technical description of the new analyzer and its optimized operational settings for detecting volatile amines via proton transfer reactions from hydronium (H3O+) or ammonium (NH ions. We show that the new prototype PTR-ToF-MS instrument is capable of monitoring rapid changes of sticky amines on the timescale of a few seconds and detects atmospheric variations of amines down to single-digit parts per trillion by volume (pptv) levels. Application examples given include the real-time monitoring of (i) methylamine emitted from a Chenopodium vulvaria L. plant, (ii) small alkylamines in ambient air on site of an agricultural research center (Senter for husdyrforsøk, Ås, Norway), and (iii) an industrial amine (2-amino-2-methylpropan-1-ol, AMP) on site and downwind of a carbon dioxide (CO2) capture test center (Technology Centre Mongstad – TCM, Mongstad, Norway).
- Article
(863 KB) - Full-text XML
-
Supplement
(755 KB) - BibTeX
- EndNote
Proton-transfer-reaction mass spectrometry (PTR-MS) is widely used in atmospheric sciences for the detection of non-methane organic gases (NMOGs; de Gouw and Warneke, 2007; Hansel et al., 1995; Yuan et al., 2017). PTR-MS is a direct-injection chemical ionization mass spectrometry (CIMS) technique. Direct injection means that the sample is directly introduced into the analyzer without any pre-collection or pretreatment. The measurements are thus conducted in real time, with a frequency of up to 10 Hz. In its original and still most widely used configuration, PTR-MS uses gas-phase hydronium ions (H3O+) as chemical ionization (CI) reagent ions. H3O+ ions react with most NMOGs in non-dissociative proton transfer reactions (Hunt and Ryan, 1972; Lindinger et al., 1998). PTR-MS distinguishes itself from other CIMS techniques in the way that the ionization is effected in a small flow tube reactor, at reduced pressure (1–4 mbar) and under the action of an electrostatic field (40–70 V cm−1). The collisional drift of the ions at high velocities prevents them from getting hydrated and limits their residence time in the flow tube. Because of the presence of an electrostatic field, the flow tube reactor is commonly referred to as the drift tube (DT). While the original PTR-MS instrument was equipped with a quadrupole mass spectrometer (QMS), state-of-the-art analyzers include a time-of-flight mass spectrometer (ToF-MS).
Currently, atmospheric composition researchers mainly use two types of PTR-ToF-MS instruments, which are manufactured by two different producers. The instrument produced by Ionicon Analytik (Innsbruck, Austria) uses a hollow cathode glow discharge (HCGD) for producing H3O+ ions (Hansel et al., 1995; Müller et al., 2020). More details about this ion source are provided in the “Instrument description” section. In the conventional and widely used Ionicon instruments, the DT is composed of a stack of stainless steel (SS) rings, which are separated by insulating Teflon spacers. In the instrument produced by Tofwerk (Thun, Switzerland), also known as the VOCUS PTR-TOF or simply as “the VOCUS”, H3O+ ions are formed in a water plasma burning between two conical electrodes (Krechmer et al., 2018). In the VOCUS analyzer, the DT consists of a resistive glass tube surrounded by cylindrical quadrupole rods. The latter generate a focusing quadrupole field within the DT, which is thus commonly referred to as a focusing ion–molecule reactor (FIMR).
Herein, we report on the development and application of a new prototype PTR-ToF-MS instrument that hybridizes the two commercial PTR-ToF-MS designs, combining an FIMR with an HCGD ion source. In a conventional stacked-ring DT, the ion swarm broadens as it drifts through the reactor, and only a fraction of the ions are sampled through the exit aperture towards the mass analyzer. This loss in sensitivity can partly be compensated for by placing an ion funnel at the end of the DT (Barber et al., 2012). Such an ion funnel is routinely installed nowadays in most Ionicon analyzers (Pugliese et al., 2020). In the FIMR, a focusing quadrupole field acts over the entire drift region, which minimizes ion losses and boosts the instrument sensitivity (103−104 cps ppbv−1; Krechmer et al., 2018). We thus decided to build an instrument based on the FIMR design proposed by Krechmer et al. (2018). Since the VOCUS ion source was not yet commercially available when we conceived the new instrument, we opted to couple the FIMR with the existing HCGD ion source.
We will describe the new prototype PTR-ToF-MS instrument in the “Instrument description” section of this paper. In the “Results and discussion” section, we will describe its performance for the detection of volatile amines and show laboratory and field data. Amines were chosen as target analytes because of the increasing interest of the atmospheric chemistry community in this class of compounds (Ge et al., 2011). This interest is mainly driven by the important role that amines play in new particle formation (e.g., Almeida et al., 2013). Amines are also being emitted to the atmosphere from carbon dioxide (CO2) capture plants. Since the slip of amines from CO2 capture plants may result in the atmospheric formation of harmful substances (e.g., nitrosamines and nitramines; Nielsen et al., 2012), amines are receiving increased interest from the air quality and health community. Atmospheric amines are however particularly challenging to measure. This is on the one hand caused by the fact that atmospheric levels are usually extremely low, requiring analyzers to be able to detect single-digit parts per trillion by volume (pptv) levels. On the other hand, amines are also known to be “sticky”, meaning that they easily adsorb onto surfaces in the analyzer and in the inlet lines. Direct-injection CIMS is thus the method of choice for measuring pptv levels amines in the atmosphere in real time, and a series of instruments have recently been developed for detecting amines (Hanson et al., 2011; Lee, 2022; Pfeifer et al., 2020; Sellegri et al., 2005; Wang et al., 2020; Yao et al., 2016; You et al., 2014; Yu and Lee, 2012; Zheng et al., 2015). We will herein show that our new PTR-ToF-MS analyzer is also capable of detecting atmospheric amines down to single-digit pptv levels. We will furthermore demonstrate that our new instrument only has minor memory effects and is thus capable of monitoring changes of amines in the air on the timescale of a few seconds.
2.1 Instrument description
The new prototype PTR-ToF-MS analyzer that combines a conventional HCGD ion source with the more recently developed FIMR is shown in Fig. 1.
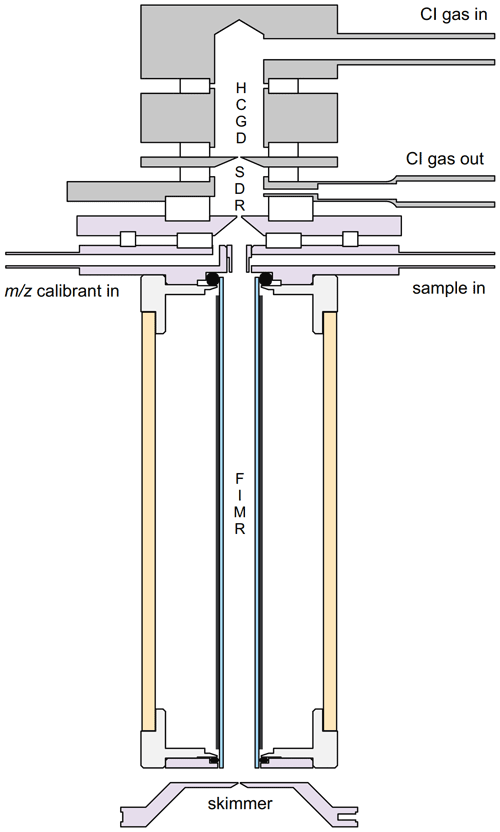
Figure 1Schematic drawing of the new prototype PTR-ToF-MS analyzer. The instrument consists of a hollow cathode glow discharge (HCGD) ion source, a source drift region (SDR) and a focusing ion–molecule reactor (FIMR), which is a resistive glass drift tube surrounded by cylindrical quadrupole rods.
The HCGD ion source and the processes occurring therein have been described in detail in the original PTR-MS publication (Hansel et al., 1995). Only minor modifications have been implemented since then, and the current configuration has been described by Müller et al. (2020). Only the essentials are thus outlined here (Fig. 1). A DC water plasma is generated inside a plasma source consisting of a hollow cylinder anode, a hollow cylinder cathode and an anode lens with an exit orifice. The entire effluent from this plasma source, which includes ions, electrons, radicals and mostly neutral H2O molecules, is introduced into a hollow cylinder electrode, which is commonly referred to as the “source drift region” or “source drift ring” (SDR). The SDR is a key component of the ion source setup because it is mostly here that O+, H+, H, OH+ and H2O+ cations react with H2O molecules to form H3O+ ions. Only ions (≥98 % H3O+, with O and NO+ making up for the rest) are extracted via the SDR exit lens and injected into the drift region. It is also in the SDR that free electrons recombine with cations and that OH• and other radical species are destroyed on SS surfaces. Importantly, the water vapor and other neutral plasma effluents are pumped off from the SDR and thus not brought into contact with the sample gas that is introduced into the instrument further downstream. This is a key design feature, which distinguishes the HCGD ion source from the commercial VOCUS ion source, in which the entire plasma effluent is introduced into the FIMR. From the available literature on the VOCUS ion source, it is not clear if the conversion to H3O+ ions is complete and if all radicals are quenched when the sample gas first interacts with the outflow from the ion source. We think that this should be investigated further, as non-protonated analyte molecules may be formed, and highly reactive NMOGs may be oxidized in the FIMR. The latter would result in important positive measurement artifacts for oxidized and highly oxidized compounds, even if only a very small and unnoticeable fraction (≤0.1 %) of highly reactive NMOGs were oxidized in the FIMR.
The entire HCGD ion source setup can be heated. Five parameters can be set in the HCGD ion source: (i) the source gas flow (Φsource), (ii) the source temperature (Tsource), (iii) the plasma current (Iplasma), (iv) the voltage between the anode lens and the SDR (USDR,in) and (v) the voltage between the SDR and the SDR exit lens (USDR,out). The specific settings used for the measurements reported herein will be reported below. The HCGD ion source has also been used for generating other types of CI reagent ions such as Oor NO+ (Jordan et al., 2009) and NH (Müller et al., 2020; Zhu et al., 2018). We only operated the new instrument in the H3O+ mode and in the NH mode, since both of these CI reagent ions can be used for detecting amines via non-dissociative proton transfer reactions (Zhu et al., 2018).
The FIMR has been described in detail by Krechmer et al. (2018). We also use a 10 cm long resistive glass tube but with a slightly smaller diameter (8 mm o.d., 6 mm i.d.). Four operational parameters can be set in the FIMR: the frequency (fFIMR) and peak-to-peak amplitude voltage (Vpp,FIMR) of the radio frequency (RF) applied on the quadrupole rods, the DC voltage applied across the ends of the glass tube (Udrift) and the pressure (pdrift) in the vacuum chamber in which the resistive glass tube is placed. The sample gas is introduced into the FIMR via a custom-made passivated (Sulfinert®, Restek, Bellefonte, PA, USA) gas inlet lens (GIL). The GIL is placed between the SDR exit lens and the FIMR. Two additional voltages can be set relative to the GIL: (i) the voltage between the SDR exit lens and the GIL (UGIL,in) and (ii) the voltage between the GIL and the upper end of the FIMR (UGIL,out). The GIL is connected to a 15 cm long in. o.d. Teflon PFA tube, which includes a T piece to branch off part of the inlet flow into a digital pressure controller (Bronkhorst High-Tech B.V., Ruurlo, the Netherlands). This device keeps the pressure in the Teflon inlet tube (pinlet) (and thereby also pdrift) constant. The inlet line and gas inlet lens can be heated (Tinlet), which indirectly heats the FIMR. The GIL has a second port that is connected to a capillary leak through which a mass axis calibration gas (1,4-diiodobenzene; Sigma-Aldrich, Oslo, Norway) diffuses into the FIMR. The sample gas is pumped off through a 1 mm gap between the lower end of the FIMR and the skimmer lens at the entrance of the mass spectrometer. The skimmer is grounded, and the voltage between the lower end of the FIMR and the skimmer (UFIMR,out) can be set. All specific settings used for the measurements reported herein will be reported below.
Our key strategy for reducing the instrumental time response was to maximize the flow through the low-pressure flow reactor. A dry multi-stage Roots pump (ACP40; Pfeiffer Vacuum, Asslar, Germany) pumps up to 0.80 standard liters per minute (slpm; “standard” herein means referenced to a temperature of 273.15 K and a pressure of 101 325 Pa) through the FIMR. For the measurements presented herein, we set the flow rate to 0.55 slpm, which is a factor of 5 higher than in commercial VOCUS instruments (Krechmer et al., 2018; Wang et al., 2020). We observed that increasing the reactor flow from 0.05 to 0.50 slpm reduced the decay time of a 20 pptv trimethylamine (TMA) signal from ∼60 to ∼5 s.
The mass analyzer used in our instrument is a quadrupole ion guide orthogonal acceleration reflectron ToF-MS (model HTOF; Tofwerk, Thun, Switzerland). The quadrupole ion guide (commonly referred to as the big segmented quadrupole, BSQ) was operated at an RF of 4.4 MHz and an RF amplitude between 300 and 320 V. It is important to note that we operate the BSQ at relatively high RFs to avoid strong mass discrimination effects in the 30 to 60 region, which is typically observed for commercial VOCUS analyzers (Krechmer et al., 2018; Wang et al., 2020).
2.2 Laboratory test
The first application test was carried out in the laboratory. We cultivated a stinking goosefoot (Chenopodium vulvaria L.) plant, which is a weed covered by minuscule bladder hairs. Upon mechanical stress, these bladder hairs release TMA. The plant was placed in our laboratory next to the analyzer and mechanically stressed. The evolving TMA puff was monitored in real time by our new prototype PTR-ToF-MS instrument.
2.3 Field tests
A first field test was carried out on campus of a research center for animal husbandry (Senter for husdyrforsøk – Livestock Production Research Centre, Norwegian University of Life Sciences, Ås, Norway). The prototype PTR-ToF-MS analyzer was installed in a temperature-controlled enclosed car trailer. The trailer was placed close to large manure tanks where the animal excrement from the entire site is collected. The aim was to monitor atmospheric amines, with a focus on methylamine (MMA), dimethylamine (DMA) and TMA. Air was sampled through a hole in the lateral wall of the trailer, using a 130 cm long, heated (100 ∘C), Sulfinert®-passivated, in. o.d. SS tube pumped at a flow rate of 25 slpm. The instrument subsampled from this main inlet flow. A flow of 2.5 standard cubic centimeters per minute (sccm) of 1 % ammonia (NH3) in nitrogen (BOC Ltd., UK) was continuously added at the tip of the main inlet for chemically passivating all wetted surfaces in the inlet and in the instrument itself (Roscioli et al., 2015; Zhu et al., 2017). Excess ammonia is known to displace amines adsorbed onto surface sites (Ongwandee et al., 2005). The instrumental background was measured every 12 h for 25 min by overflowing the instrument subsampling flow with ambient air that had passed through a heated (350 ∘C) Pt–Pd catalyst. We have shown in previous work that MMA, DMA and TMA are efficiently detected in their protonated form if NH ions are used as CI reagent ions in PTR-MS instruments (Zhu et al., 2018). In this first field study, we thus operated the HCGD ion source in the NH mode with the following settings: Φsource=6 sccm (1 %–3 % NH3 in N2, Nippon Gases Norge AS, Oslo, Norway) and Isource=4 mA; Tsource=100 ∘C. Ions with a relative abundance >1 % of NH were H3O+ (∼5 %), NO+ (∼3 %), O (∼3 %) and NH(NH3) (∼1 %; Fig. S1 in the Supplement). For quantification, we estimated that NH and H3O+ have a 50 % lower detection efficiency than the other three ion species (due to increased mass discrimination of ions with ). The operational parameters of the instrument were as follows: Tinlet=100 ∘C, V, V, V, V, Udrift=450 V, V, pdrift=1.95 mbar, fFIMR=5.5 MHz and V. The FIMR was operated at relatively high RFs to avoid strong mass discrimination effects in the 30 to 60 region. Calibrations were performed in the field using a liquid calibration unit (LCU) (LCU-a, Ionimed Analytik GmbH, Innsbruck; Fischer et al., 2013). An aqueous standard solution of MMA, DMA and TMA was prepared by diluting known volumes of commercial solutions of MMA (40±3 % wt. in H2O), DMA (40±2 % wt. in H2O) and TMA (45±3.5 % wt. in H2O) with Type 1 Milli-Q® water. In the LCU, the standard solution was nebulized/evaporated at a known liquid flow rate (2–10 µL min−1) into a known flow (typically 1 slpm) of NMOG-free air. The accuracy of the MMA, DMA and TMA calibration is conservatively estimated to be ±15 %.
Two additional field tests were conducted at and near the Technology Centre Mongstad (TCM; Mongstad, Norway). TCM is one of the world's largest test centers for amine-based CO2 capture (de Koeijer et al., 2011). The measurements were carried out during a test campaign with a solvent containing 2-amino-2-methylpropan-1-ol (AMP; Languille et al., 2021). The instrument was calibrated with the LCU using an aqueous solution of AMP, which was prepared by diluting a weighted amount of the pure compound (≥99 %; Sigma-Aldrich, Oslo, Norway) in Type 1 Milli-Q® water. The accuracy of the AMP calibration is conservatively estimated to be ±15 %. The first test was carried out on the premises of TCM to demonstrate that the new instrument is capable of detecting solvent leaks in real time. For this purpose, a gas mixture containing 25 ppmv of AMP was deliberately released into the air at approximately 1 m distance from the instrument inlet using a HovaCAL® (model digital 211-MF; IAS GmbH, Oberursel, Germany) gas generator. The second test was to demonstrate that the new and highly sensitive PTR-ToF-MS analyzer is capable of detecting single-digit pptv levels of amines in the downwind atmosphere of a CO2 capture facility. For this purpose, the instrument trailer was placed ∼4.4 km north of TCM during a 1-week period with predominantly southerly winds. The measurements took place when ∼1 ppmv of AMP of TCM was steadily emitted from TCM. For these measurements, the prototype PTR-ToF-MS analyzer was operated in the conventional H3O+ mode. Some operational parameters were thus modified as compared to the first field campaign: Φsource=6 sccm (H2O), Isource=4.5 mA, V, V; V and Udrift=400 V. The instrumental background was measured once a day for 20 min by overflowing the instrument subsampling flow with ambient air that had passed through a heated (350 ∘C) Pt–Pd catalyst. The data from the first 5 min of zeroing were discarded because of signal tailing.
3.1 Laboratory testing: detection of biogenic amines
Figure 2 shows the time evolution of TMA in laboratory air when a Chenopodium vulvaria L. plant was mechanically stressed by tipping a leaf with a pen. The stress resulted in a burst of TMA, and the new prototype PTR-ToF-MS instrument detected a short-term spike in TMA with a peak width (full width half maximum, FWHM) of 16 s. This was our first experimental evidence that the new prototype instrument is capable of monitoring rapid changes of amine levels in the air on the timescale of a few seconds. The TMA volume mixing ratio did not drop to pre-spike levels because the amine was released into and sampled from laboratory air. We did not make any further attempts to quantify TMA emission rates.
3.2 Field testing: detection of agricultural amines
After the first successful laboratory tests, the instrument was taken to the field. The goal was to measure small alkylamines in ambient air on campus of an agricultural research center. As mentioned in the “Instrument description” section, the prototype PTR-ToF-MS instrument was operated in the NH mode.
Figure 3 displays the calibration curves for MMA, DMA and TMA, as obtained from a calibration carried out in the field. The calibration curves show the normalized ion signal response (in normalized counts per second, ncps) as a function of amine volume mixing ratios in the 0 to 10 ppbv range. The signals were normalized to the average NH signal (measured on the 15NH isotope signal at 19.031) observed over the course of the experiment. The three amines were detected in their protonated form at 32.049, 46.065 and 60.081, respectively. The only additional signals observed were 44.049 (2.5 %) and 58.065 (4.0 %) for DMA and TMA, respectively. H2 ejection is a frequently observed minor channel in the CI of amines (Harrison, 1992). No adduct ions were detected due to the high collisional energy of the ions in the FIMR. The protonated amines were detected at a mass-resolving power () of 3700, 4100 and 4250, respectively. The instrumental response factors (sensitivities) for MMA, DMA and TMA were 114, 156 and 126 ncps ppbv−1, respectively. The instrumental response did not exhibit any humidity dependence (<3 % analyte ion signal change over the range of typical ambient humidities). The relative precision (1σ) at the lowest calibration mixing ratio (∼2 ppbv) was 4.3 %, 2.2 % and 4.3 % for MMA, DMA and TMA, respectively. The offset in the calibration curves mainly originated in a constant chemical background in the calibration device (LCU) from previous calibrations. The data shown in Fig. 3 were thus not used for deriving instrumental detection limits.
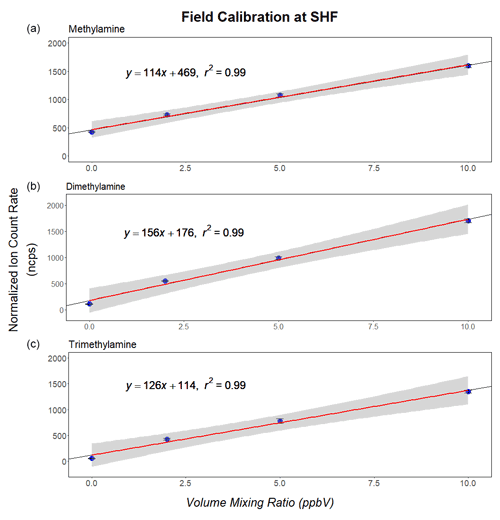
Figure 3Calibration curves for methylamine (a), dimethylamine (b) and trimethylamine (c). The PTR-ToF-MS instrument was operated in the NH mode (see text).
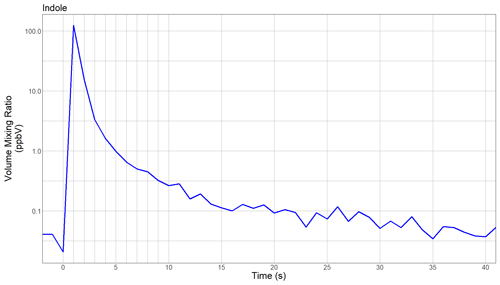
Figure 4Time evolution of the observed indole volume mixing ratio (VMR) when the headspace from a vial containing indole was shortly blown towards the PTR-ToF-MS instrument in its field configuration.
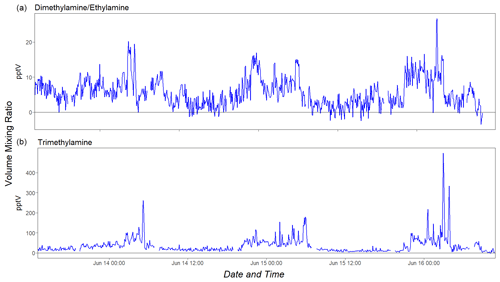
Figure 5Time series (5 min averages) of dimethylamine/ethylamine (a) and trimethylamine (b) as observed in ambient air on site of an agricultural research center.
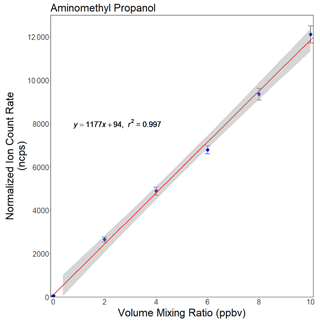
Figure 6Calibration curve for 2-amino-2-methylpropan-1-ol (AMP). The PTR-ToF-MS instrument was operated in the H3O+ mode (see text).
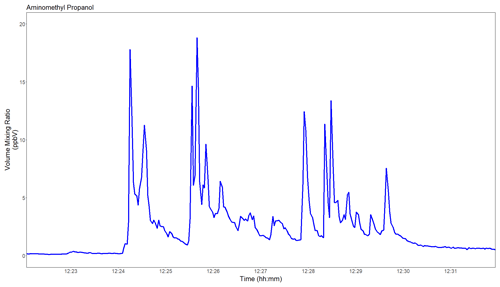
Figure 7Time series (1 Hz) of 2-amino-2-methylpropan-1-ol (AMP) as measured when ∼25 ppmv of this industrial amine was deliberately released into the air at approximately 1 m distance from the instrument inlet.
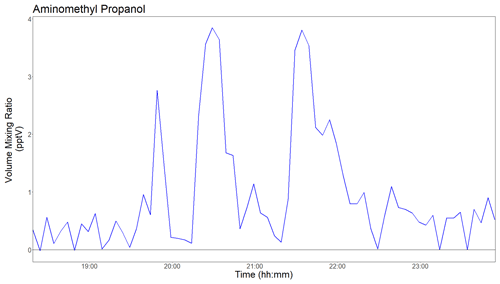
Figure 8Time series (5 min averages) of 2-amino-2-methylpropan-1-ol (AMP) as measured at Sande, 4.4 km downwind of the TCM CO2 capture plant. A steady level of ∼1 ppmv of AMP was released from the stack during the measurement period, which resulted in single-digit pptv enhancements of AMP at the downwind site.
The instrumental response factors of the prototype PTR-ToF-MS analyzer are 1–2 orders of magnitude lower than those reported for commercial VOCUS analyzers (Krechmer et al., 2018; Wang et al., 2020). This is a result of a combination of various factors. First, we generally observe lower instrumental sensitivities when operating PTR-MS instruments in the NH mode (Zhu et al., 2018). Second, we set the upper limit in the TOF-MS to ∼500. Reducing the upper limit to 100 would increase the sensitivity by a factor of 2.2 (higher duty cycle). Third and most importantly, our prototype instrument has a relatively large gap between the FIMR and the skimmer (to increase the flow through the reactor and thereby minimize memory effects), which results in increased ion losses at the end of the FIMR. Fourth, we apply a relatively low RF amplitude to the FIMR because at higher Vpp, O ions efficiently formed within the FIMR. A high O signal on 31.989 severely compromises the detection of MMA at 32.049. Fifth, we also apply a relatively low RF amplitude to the BSQ to avoid strong mass discrimination effects in the 32 to 60 region. The small spread in the MMA, DMA and TMA sensitivity values confirms that no significant mass discrimination occurred in the range of interest.
Prior to starting the measurements, we carried out another field test. Indole was among the analytes of interest for this field study (even though it was later only sporadically detected at very low levels), and laboratory tests had revealed that this amine is particularly sticky. The headspace from a vial containing indole was thus shortly blown towards the instrument inlet, and the protonated indole signal at 118.065 was monitored at 1 s time resolution. Figure 4 shows the time series of indole as observed during this experiment. The initial volume mixing ratio on the order of 100 ppbv decreased by 2 orders of magnitude within 4 s, and background levels were reached within ∼40 s. We are fully aware that this instrument test deviates from the formal experimental procedure for determining an instrumental response time. However, these data do show the performance of the analyzer under field conditions and allow us to conclude that the new prototype instrument is indeed capable of monitoring rapid changes of sticky amines on the timescale of a few seconds.
Figure 5 shows the time series (5 min averages) of DMA and TMA as measured in ambient air on site at the agricultural research center over a period of 3 d. The instrumental background was stable for both DMA and TMA (see Fig. S2), which resulted in a detection limit (3σ, 5 min signal integration) of ∼5 pptv for these two amines. It was not possible to detect protonated MMA ( 32.049) because of the strong mass spectral interference from the adjacent O peak ( 31.989). We subtracted the O contribution, but the resulting detection limit (3σ, 5 min signal integration) was ∼170 pptv. It is important to note that direct-injection CIMS methods do not resolve isomeric species. DMA only has one additional isomer (ethylamine, EA), while TMA has three isomers (ethylmethylamine, propylamine and isopropylamine). Previous work (Schade and Crutzen, 1995, and references therein) found trace levels of both EA and DMA in husbandry emissions, which is why we assign the 46.065 ion signal to both of these compounds. The same literature reports that TMA is the dominant C3 isomer, which is why we assign the 60.081 signal to TMA only. We observed a clear diurnal pattern in the time profile of both species, with maxima occurring during nighttime when the planetary boundary layer is low.
3.3 Field testing: detection of an industrial amine
The field tests at and near the CO2 capture plant were carried out with the instrument being operated in the conventional H3O+ mode. The calibration curve (Fig. 6) shows the 90.091 normalized ion signal response (in ncps, normalized to the average H3O H3O+(H2O) signal observed over the course of the calibration experiment) as a function of the AMP volume mixing ratio. The mass-resolving power () for this ion signal was 3700.
The calibration data show that the prototype PTR-ToF-MS instrument generated a signal response >103 ncps ppbv−1. This is still on the lower end of what has been reported for commercial VOCUS analyzers. As mentioned above, this is mainly due to ion losses in the relatively large gap between the FIMR and the skimmer, which is needed for increasing the FIMR flow. The relative precision (1σ) at the lowest calibration mixing ratio (∼2 ppbv) was ±4.2 % for AMP. The offset in the calibration curve was again caused by a constant chemical background in the LCU calibration device and not in the instrument itself. The data shown in Fig. 6 were thus not used for deriving the instrumental detection limit for AMP.
The first test carried out at TCM was to demonstrate that the new instrument is capable of detecting amine solvent leaks in real time. A gas mixture containing ∼25 ppmv AMP was deliberately released into the air at approximately 1 m distance from the instrument inlet. The test was carried out during strong and turbulent wind conditions, which are typical for the Norwegian west coast. As seen in Fig. 7, single- to double-digit parts per billion per volume (ppbv) levels of AMP were observed in real time (1 Hz). Notably, the instrument response was immediate, and the signal quickly returned to baseline levels once the artificial leak was closed.
The second test was to demonstrate that the new PTR-ToF-MS analyzer is capable of detecting ultra-trace levels of industrial amines in the atmosphere. During the measurement period, a steady level of ∼1 ppmv AMP was released to the atmosphere from TCM, while the PTR-ToF-MS instrument sampled atmospheric air ∼4.4 km downwind. Figure 8 shows 5 min average AMP data collected when the TCM plume crossed the measurement site. The instrumental background was low and stable (0.6±0.2 pptv) throughout the measurement period (see Fig. S3), which resulted in a detection limit (3σ, 5 min signal integration) of ∼0.5 pptv for AMP. As seen in Fig. 8, at 5 min signal integration variations <1 pptv can be observed.
The PTR-MS technique was developed in the mid-1990ies at the University of Innsbruck. Initial method improvements focused on using different mass analyzers (e.g., Blake et al., 2004; Prazeller et al., 2003). The ion source and drift tube only underwent minor geometrical changes over a period of 2 decades. The past 5 years have, however, seen major developments in the drift tube and ion source design (e.g., Breitenlechner et al., 2017; Krechmer et al., 2018). We have herein presented one additional instrument variation, in which the well-characterized and “clean” HCGD ion source originally described by Hansel et al. (1995) is combined with the recently developed and highly sensitive FIMR design by Krechmer et al. (2018). We have shown data that demonstrate the capabilities of this new instrument in monitoring atmospheric amines at single-digit pptv levels. We have also shown that the new instrument only has minor memory effects for sticky amines, making it capable of monitoring rapid changes of amine levels in the air on the timescale of a few seconds. Further work is however required for achieving a time response of 1 s or less. This could be achieved by further optimizing the gas inlet or by heating the FIMR to ≥100 ∘C. We have also made an argument that maximizing the instrument sensitivity should not be the only focus of PTR-MS instrument development and optimization. Minimizing potential neutral and ionic side reactions in the DT, reducing sample memory effects and eliminating mass discrimination effects over the range of interest are important for generating easily interpretable and quantifiable mass spectra. This is particularly important for the nonexpert user. The PTR-MS method is being rapidly developed in various directions, and different types of PTR-MS instruments and operation modes are being used nowadays for different types of applications. The PTR-ToF-MS presented herein has been optimized for the detection of atmospheric amines, which are currently in the research focus of our group. In future work, we will show how we have used the new prototype PTR-ToF-MS instrument for quantifying amine emission rates from animal husbandry and for investigating the atmospheric impact of amine emissions from CO2 capture.
The data used in this paper are available from the corresponding author upon request.
The supplement related to this article is available online at: https://doi.org/10.5194/amt-15-6297-2022-supplement.
TM and AW conceived the instrument. TM and AH built and optimized the instrument. AH carried out the lab and field measurements, with help from EES and logistical support from TM. AH analyzed all data. AH drafted the manuscript. AW wrote the final paper. All authors commented on and accepted the final version of the paper.
The contact author has declared that none of the authors has any competing interests.
Publisher’s note: Copernicus Publications remains neutral with regard to jurisdictional claims in published maps and institutional affiliations.
We thank the Senter for husdyrforsøk (SHF) at the Norwegian University of Life Sciences (NMBU) for hosting our new prototype instrument. Irma Caroline Oskam and Birgitte Mosveen from SHF are acknowledged for their hospitality and logistical support. We also thank the Technology Centre Mongstad (TCM) and in particular Audun Drageset and Øyvind Ullestad for logistical support. Baptiste Languille is acknowledged for logistical support of the field measurements at TCM. We thank the two anonymous reviewers for their constructive comments and suggestions. Special thanks go to Marc Gonin from Tofwerk for sharing his early ideas on quadrupole-guided resistive glass tubes and to Tofwerk for providing a prototype FIMR. We apologize that it took so long to make something of it.
Alexander Håland received a doctoral fellowship from the Centre for Biogeochemistry in the Anthropocene (CBA) at the University of Oslo. Armin Wisthaler was financially supported by the acquisition of the HTOF from the University of Oslo via his start-up grant. Technology Centre Mongstad (TCM) financially supported the measurements at and near its site through the ARM (Amine Research and Monitoring) project.
This paper was edited by Glenn Wolfe and reviewed by two anonymous referees.
Almeida, J., Schobesberger, S., Kürten, A., Ortega, I. K., Kupiainen-Määttä, O., Praplan, A. P., Adamov, A., Amorim, A., Bianchi, F., Breitenlechner, M., David, A., Dommen, J., Donahue, N. M., Downard, A., Dunne, E., Duplissy, J., Ehrhart, S., Flagan, R. C., Franchin, A., Guida, R., Hakala, J., Hansel, A., Heinritzi, M., Henschel, H., Jokinen, T., Junninen, H., Kajos, M., Kangasluoma, J., Keskinen, H., Kupc, A., Kurtén, T., Kvashin, A. N., Laaksonen, A., Lehtipalo, K., Leiminger, M., Leppä, J., Loukonen, V., Makhmutov, V., Mathot, S., Mcgrath, M. J., Nieminen, T., Olenius, T., Onnela, A., Petäjä, T., Riccobono, F., Riipinen, I., Rissanen, M., Rondo, L., Ruuskanen, T., Santos, F. D., Sarnela, N., Schallhart, S., Schnitzhofer, R., Seinfeld, J. H., Simon, M., Sipilä, M., Stozhkov, Y., Stratmann, F., Tomé, A., Tröstl, J., Tsagkogeorgas, G., Vaattovaara, P., Viisanen, Y., Virtanen, A., Vrtala, A., Wagner, P. E., Weingartner, E., Wex, H., Williamson, C., Wimmer, D., Ye, P., Yli-Juuti, T., Carslaw, K. S., Kulmala, M., Curtius, J., Baltensperger, U., Worsnop, D. R., Vehkamäki, H., and Kirkby, J.: Molecular understanding of sulphuric acid–amine particle nucleation in the atmosphere, Nature, 502, 359–363, https://doi.org/10.1038/nature12663, 2013.
Barber, S., Blake, R. S., White, I. R., Monks, P. S., Reich, F., Mullock, S., and Ellis, A. M.: Increased Sensitivity in Proton Transfer Reaction Mass Spectrometry by Incorporation of a Radio Frequency Ion Funnel, Anal. Chem., 84, 5387–5391, https://doi.org/10.1021/ac300894t, 2012.
Blake, R. S., Whyte, C., Hughes, C. O., Ellis, A. M., and Monks, P. S.: Demonstration of Proton-Transfer Reaction Time-of-Flight Mass Spectrometry for Real-Time Analysis of Trace Volatile Organic Compounds, Anal. Chem., 76, 3841–3845, https://doi.org/10.1021/ac0498260, 2004.
Breitenlechner, M., Fischer, L., Hainer, M., Heinritzi, M., Curtius, J., and Hansel, A.: PTR3: An Instrument for Studying the Lifecycle of Reactive Organic Carbon in the Atmosphere, Anal. Chem., 89, 5824–5831, https://doi.org/10.1021/acs.analchem.6b05110, 2017.
de Gouw, J. and Warneke, C.: Measurements of volatile organic compounds in the earth's atmosphere using proton-transfer-reaction mass spectrometry, Mass Spectrom. Rev., 26, 223–257, https://doi.org/10.1002/mas.20119, 2007.
de Koeijer, G., Enge, Y., Sanden, K., Graff, O. F., Falk-Pedersen, O., Amundsen, T., and Overå, S.: CO2 Technology Centre Mongstad – Design, functionality and emissions of the amine plant, Energy Proced., 4, 1207–1213, https://doi.org/10.1016/j.egypro.2011.01.175, 2011.
Fischer, L., Klinger, A., Herbig, J., Winkler, K., Gutmann, R., and Hansel, A.: The LCU: Versatile Trace Gas Calibration, in 6th International Conference on Proton Transfer Reaction Mass Spectrometry and its Applications, edited by: Hansel, A. and Dunkl, J., Innsbruck University Press, Innsbruck, 192–195, https://openpub.fmach.it/retrieve/handle/10449/23075/9394/ptrms_2013.pdf#page=193 (last access: 31 October 2022), 2013.
Ge, X., Wexler, A. S. and Clegg, S. L.: Atmospheric amines – Part I. A review, Atmos. Environ., 45, 524–546, https://doi.org/10.1016/j.atmosenv.2010.10.012, 2011.
Hansel, A., Jordan, A., Holzinger, R., Prazeller, P., Vogel, W., and Lindinger, W.: Proton transfer reaction mass spectrometry: on-line trace gas analysis at the ppb level, Int. J. Mass Spectrom. Ion Process., 149–150, 609–619, https://doi.org/10.1016/0168-1176(95)04294-U, 1995.
Hanson, D. R., Mcmurry, P. H., Jiang, J., Tanner, D., and Huey, L. G.: Ambient Pressure Proton Transfer Mass Spectrometry: Detection of Amines and Ammonia, Environ. Sci. Technol., 45, 8881–8888, https://doi.org/10.1021/es201819a, 2011.
Harrison, A. G. (Ed.): Chemical Ionization Mass Spectrometry, 2nd edn., Routledge, New York, https://doi.org/10.1201/9781315139128, 1992.
Hunt, D. F. and Ryan, J. F.: Argon-water mixtures as reagents for chemical ionization mass spectrometry, Anal. Chem., 44, 1306–1309, https://doi.org/10.1021/ac60315a024, 1972.
Jordan, A., Haidacher, S., Hanel, G., Hartungen, E., Herbig, J., Märk, L., Schottkowsky, R., Seehauser, H., Sulzer, P., and Märk, T. D.: An online ultra-high sensitivity Proton-transfer-reaction mass-spectrometer combined with switchable reagent ion capability (PTR + SRI − MS), Int. J. Mass Spectrom., 286, 32–38, https://doi.org/10.1016/J.IJMS.2009.06.006, 2009.
Krechmer, J., Lopez-Hilfiker, F. D., Koss, A. R., Hutterli, M. A., Stoermer, C., Deming, B. L., Kimmel, J. R., Warneke, C., Holzinger, R., Jayne, J. T., Worsnop, D. R., Fuhrer, K., Gonin, M., and De Gouw, J.: Evaluation of a New Reagent-Ion Source and Focusing Ion-Molecule Reactor for Use in Proton-Transfer-Reaction Mass Spectrometry, Anal. Chem., 90, 12011–12018, https://doi.org/10.1021/acs.analchem.8b02641, 2018.
Languille, B., Drageset, A., Mikoviny, T., Zardin, E., Benquet, C., Ullestad, Ø., Aronson, M., Kleppe, E. R., and Wisthaler, A.: Best practices for the measurement of 2-amino-2-methyl-1-propanol, piperazine and their degradation products in amine plant emissions, Proceedings of the 15th Greenhouse Gas Control Technologies Conference, Abu Dhabi, UAE, 15–18 March 2021, https://doi.org/10.2139/ssrn.3812339, 2021.
Lee, S.-H.: Perspective on the Recent Measurements of Reduced Nitrogen Compounds in the Atmosphere, Front. Environ. Sci., 10, 1–9, https://doi.org/10.3389/fenvs.2022.868534, 2022.
Lindinger, W., Hansel, A., and Jordan, A.: Mass Spectrometry Online monitoring of volatile organic compounds at pptv levels by means of Proton-Transfer-Reaction Mass Spectrometry (PTR-MS) Medical applications, food control and environmental research, Int. J. Mass Spectrom. Ion Process., 173, 191–241, https://doi.org/10.1016/S0168-1176(97)00281-4, 1998.
Müller, M., Piel, F., Gutmann, R., Sulzer, P., Hartungen, E., and Wisthaler, A.: A novel method for producing NH4+ reagent ions in the hollow cathode glow discharge ion source of PTR-MS instruments, Int. J. Mass Spectrom., 447, 116254, https://doi.org/10.1016/j.ijms.2019.116254, 2020.
Nielsen, C. J., Herrmann, H., and Weller, C.: Atmospheric chemistry and environmental impact of the use of amines in carbon capture and storage (CCS), Chem. Soc. Rev. Chem. Soc. Rev, 41, 6684–6704, https://doi.org/10.1039/c2cs35059a, 2012.
Ongwandee, M., Bettinger, S. S., and Morrison, G. C.: The influence of ammonia and carbon dioxide on the sorption of a basic organic pollutant to a mineral surface, Indoor Air, 15, 408–419, https://doi.org/10.1111/j.1600-0668.2005.00380.x, 2005.
Pfeifer, J., Simon, M., Heinritzi, M., Piel, F., Weitz, L., Wang, D., Granzin, M., Müller, T., Bräkling, S., Kirkby, J., Curtius, J., and Kürten, A.: Measurement of ammonia, amines and iodine compounds using protonated water cluster chemical ionization mass spectrometry, Atmos. Meas. Tech., 13, 2501–2522, https://doi.org/10.5194/amt-13-2501-2020, 2020.
Prazeller, P., Palmer, P. T., Boscaini, E., Jobson, T., and Alexander, M.: Proton transfer reaction ion trap mass spectrometer, Rapid Commun. Mass Spectrom., 17, 1593–1599, https://doi.org/10.1002/rcm.1088, 2003.
Pugliese, G., Piel, F., Trefz, P., Sulzer, P., Schubert, J. K., and Miekisch, W.: Effects of modular ion-funnel technology onto analysis of breath VOCs by means of real-time mass spectrometry, Anal. Bioanal. Chem., 412, 7131–7140, https://doi.org/10.1007/s00216-020-02846-8, 2020.
Roscioli, J. R., Zahniser, M. S., Nelson, D. D., Herndon, S. C., and Kolb, C. E.: New Approaches to Measuring Sticky Molecules: Improvement of Instrumental Response Times Using Active Passivation, J. Phys. Chem., 120, 1347–1357, https://doi.org/10.1021/acs.jpca.5b04395, 2015.
Schade, G. W. and Crutzen, P. J.: Emission of aliphatic amines from animal husbandry and their reactions: Potential source of N2O and HCN, J. Atmos. Chem., 22, 319–346, https://doi.org/10.1007/BF00696641, 1995.
Sellegri, K., Umann, B., Hanke, M., and Arnold, F.: Deployment of a ground-based CIMS apparatus for the detection of organic gases in the boreal forest during the QUEST campaign, Atmos. Chem. Phys., 5, 357–372, https://doi.org/10.5194/acp-5-357-2005, 2005.
Wang, Y., Yang, G., Lu, Y., Liu, Y., Chen, J., and Wang, L.: Detection of gaseous dimethylamine using vocus proton-transfer-reaction time-of-flight mass spectrometry, Atmos. Environ., 243, 117875, https://doi.org/10.1016/j.atmosenv.2020.117875, 2020.
Yao, L., Wang, M.-Y., Wang, X.-K., Liu, Y.-J., Chen, H.-F., Zheng, J., Nie, W., Ding, A.-J., Geng, F.-H., Wang, D.-F., Chen, J.-M., Worsnop, D. R., and Wang, L.: Detection of atmospheric gaseous amines and amides by a high-resolution time-of-flight chemical ionization mass spectrometer with protonated ethanol reagent ions, Atmos. Chem. Phys., 16, 14527–14543, https://doi.org/10.5194/acp-16-14527-2016, 2016.
You, Y., Kanawade, V. P., de Gouw, J. A., Guenther, A. B., Madronich, S., Sierra-Hernández, M. R., Lawler, M., Smith, J. N., Takahama, S., Ruggeri, G., Koss, A., Olson, K., Baumann, K., Weber, R. J., Nenes, A., Guo, H., Edgerton, E. S., Porcelli, L., Brune, W. H., Goldstein, A. H., and Lee, S.-H.: Atmospheric amines and ammonia measured with a chemical ionization mass spectrometer (CIMS), Atmos. Chem. Phys., 14, 12181–12194, https://doi.org/10.5194/acp-14-12181-2014, 2014.
Yu, H. and Lee, S.-H.: Chemical ionisation mass spectrometry for the measurement of atmospheric amines, Environ. Chem., 9, 190, https://doi.org/10.1071/EN12020, 2012.
Yuan, B., Koss, A. R., Warneke, C., Coggon, M., Sekimoto, K., and De Gouw, J. A.: Proton-Transfer-Reaction Mass Spectrometry: Applications in Atmospheric Sciences, Chem. Rev., 117, 13187–13229, https://doi.org/10.1021/acs.chemrev.7b00325, 2017.
Zheng, J., Ma, Y., Chen, M., Zhang, Q., Wang, L., Khalizov, A. F., Yao, L., Wang, Z., Wang, X., and Chen, L.: Measurement of atmospheric amines and ammonia using the high resolution time-of-flight chemical ionization mass spectrometry, Atmos. Environ., 102, 249–259, https://doi.org/10.1016/j.atmosenv.2014.12.002, 2015.
Zhu, L., Mikoviny, T., Wisthaler, A., and Nielsen, C. J.: A Sampling Line Artifact in Stack Emission Measurement of Alkanolamine-enabled Carbon Capture Facility: Surface Reaction of Amines with Formaldehyde, Energy Proc., 114, 1022–1025, https://doi.org/10.1016/j.egypro.2017.03.1247, 2017.
Zhu, L., Mikoviny, T., Kolstad Morken, A., Tan, W., and Wisthaler, A.: A compact and easy-to-use mass spectrometer for online monitoring of amines in the flue gas of a post-combustion carbon capture plant, Int. J. Greenh. Gas Control, 78, 349–353, https://doi.org/10.1016/j.ijggc.2018.09.003, 2018.